The effect of regulating the Wnt signaling pathway on the proliferation and differentiation of spermatogonial stem cells
Introduction
In recent years, cells and organ engineering research has raised new hope in infertility treatment (1,2). Spermatogonial stem cells (SSCs), niche in the basal compartment of the seminiferous tubules, is a type of germline stem cell with capacities of self-renewal and differentiation, play an important role in spermatogenesis in males (3,4). Spermatogenesis is a complex physiological process involving multiple molecules and signaling pathways, and it takes more than one month from spermatogonial stem cells to sperm formation, which through meiosis. Spermatogenic failure is a growing problem and is common among reproductive-age men, which could be caused both by diseases influence endocrine abnormalities, such as diabetes, and direct effects on the fidelity and throughput of spermatogenesis.
It is reported that many studies have documented the function of the Wnt and transforming growth factor-β (TGF-β) signaling pathways could effect many Physiological Processes, including spermatogenesis (5,6). The Wnt/β-catenin signaling pathway is involved in developmental and physiological processes, such as cell proliferation and differentiation (7,8) and can regulate and activate the production of Wnt6 protein by paracrine of Sertoli cells, and participate in the proliferation regulation of undifferentiated spermatogonia (9).
SSCs can be isolated and cultured in vitro with immunomagnetic beads. SSCs reside along the basement membrane of the seminiferous tubule in close contact with Sertoli cells. Studies have identified how Sertoli cells support SSCs. Recently reports show that Wnt proteins includes the canonical (Wnt/b-catenin and cTnnB1) and non-canonical (Wnt/Ca2+) pathways. A less studied Wnt pathway is the Wnt-JnK pathway which plays a crucial role in stem cell morphogenesis, cell proliferation, differentiation and migration (10,11).
The β-catenin complex, composed mainly of adenomatous polyposis coli (APC), axin, glycogen synthase kinase-3β (GSK-3β), and casein kinase 1, has been identified as crucial for the maintenance of the stem-like properties of stem cells and for clinic treatment resistance. In this study, we have investigated the proliferation ability and differentiation tendency of mice SSCs. Furthermore, we have studied the effect of regulating the Wnt signaling pathway on the proliferation and differentiation of SSCs, to provide a basis for the clinical application of SSCs.
We present the following article in accordance with the ARRIVE reporting checklist (available at http://dx.doi.org/10.21037/atm-20-5321).
Methods
Isolation and culture of SSCs
SPF C57BL/6 male mice (5–10 days) were purchased from Nanjing Slack Laboratory Animal Co., Ltd. The mice were put on a 12-hour dark/light cycle with chow and water provided on time. SSCs were collected from the mice and purified according to an existing protocol (12,13). In brief, the mice were killed by carbon dioxide suffocation. The testis was collected under aseptic conditions, then the seminiferous tubules were washed five times with phosphate-buffered saline (PBS). Following two-step enzymatic digestion as described by Ko et al. [2010] (14) the suspensions of testicular cells were collected. THY1-positive cells were enriched by magnetic activated cell separation (MACS). Cells were re-suspended in stem cell culture medium (Invitrogen, CA, USA), supplemented with 10% stem cell fetal bovine serum (FBS), 5% of 50 µg/mL penicillin, and streptomycin, and seeded at a density of 1×106 cells in 25 cm2 cells culture flasks. They were maintained at 37 °C in a 95% humidified atmosphere and 5% CO2 (Thermol, RBT, Germany). The medium was changed 4 days after seeding, then every 5 days until the cells reached approximately 85% confluence. The cells were passaged by 0.25% pancreatic enzyme. Cells of passages 3–9 were used for experiments. All animal experiments were performed in accordance with the guidelines for animal care and the protocol was approved by the Committee on the Ethics of Animal Experiments of Jiangsu Provincial Center for Disease Prevention and Control (approval no. JSJK/JL-161).
Surface molecular phenotype analysis of SSCs
The molecular phenotype of the mice SSCs was identified by flow cytometry (FACSCalibur, BD, NY, USA). Expression of the surface markers CDH1, ID4, NAN0S3, CD44, CD31 and CD45 were detected. 1×106 SSCs (Passage 3 and 10) were incubated with non-fluorescent labeling primary antibodies (rabbit anti-mouse CDH1, ID4, NAN0S3, CD44, CD31 and CD45) for 2 hours at room temperature. Then, cells were incubated by a secondary antibody [goat anti-rabbit Alexa Fluor 488 (Invitrogen Life Science, NY, USA)] for 1 hour at room temperature in darkness. SSCs were washed 3 times by PBS and detected using flow cytometry (BD, NJ, USA). Data were analysed using Paint-A-Gate and FlowJo software (TreeStar Inc. San Carlos, CA).
The interference sequence siRNA of the Wnt signaling pathway key molecular Axin gene
The siRNA-expressing construct of the Axin gene was generated as follows: according to the Axin gene sequence (NCBI: nm_009733), Axin gene siRNA interference sequences were designed by Ambion siRNA Target Finder software, and 5'-TCCCACTTTGAATGAGGATGAACTCGAG TTCATCCTCATTCAAAGTGGG TTTTTTC-3' was selected, chemically synthesized, annealed, and digested with EcoR I/XhoI, and ligated into the same restriction sites in the green fluorescent protein (GFP)-labeled lentivirus vector pGC-LV expression vector (GV118). DNA sequencing was applied to identify the transformant. A random siRNA sequence (TTCTCCGAACGTGTCACGT) was used as a control for Axin siRNA.
Lenti-NC and Lenti-Axin-si virus were amplified by 293T cells with ViraPower Packaging Mix and Lipofectamine 2000 reagent, according to manufacturer instructions. In brief, 293T cells were transfected with Lenti-Axin-si virus. 48 hours after transfection, the supernatant was collected and passed through 0.45 µm filters and concentrated. The viral supernatant was added into target SSCs cells at multiplicity of infection (10, 100, 200 MOI) with 5 g/mL polybrene to obtain stable Lenti-Axin-si virus transfected cells. Transfection positive cells were detected by fluorescence microscopy. Expression of the Axin protein was detected by Western-blotting.
Cell viability and proliferation after Lenti-Axin-si transfection was detected by MTT assay
MTT [3-(4,5-Dimethylthiazol-2-yl)-2,5-diphenyltetrazolium bromide] assay was performed from the first day to the tenth day after Lenti-Axin-siRNA virus transfection. In brief, the SSCs were cultured in 96-well plates. Cells were incubated with 25 µL MTT solution (5 mg/mL) for 4 hours at room temperature. Supernatant was discarded and 100 L DMSO was added to dissolve the formazan precipitate for half an hour at 37 °C. Optical density was then measured at 570 nm with an automatic microplate spectrophotometer (SpectraMax 190, Sunnyvale, USA).
Effect on SSCs differentiation by the Wnt/β-catenin signaling pathway
SSCs grouping
Cultured SSCs were divided into six groups: control group (normal SSCs), SSCs + TGF-β group (TGF-β 0.5 ng/mL was added to the culture medium to induce SSCs to differentiate into fibroblasts), SSCs + DKK1 group (positive control, Wnt/β-catenin signaling pathway inhibitor DKK1 20 ng/mL was added to the culture medium), SSCs + Axin-RNAi group (Wnt/β-Catenin signal pathway specific interference virus Axin-RNAi 10−3 was added to the culture medium to decrease Axin expression to inhibit the Wnt signal pathway), SSCs + TGF-β + DKK1 group (0.5 ng/mL TGF-β and 20 ng/mL DKK1 were both added to the culture medium), SSCs + TGF-β + Axin RNAi group (0.5 ng/mL TGF-β and Axin-RNAi 10−3 were both added to the culture medium).
Induce SSCs differentiate into fibroblasts
TGF-β was added to the culture medium to induce SSCs to differentiate into fibroblasts. Second to 5th generation mesenchymal stem cells were chosen for observation. Cells were subcultured in low glucose DMEM with 10% FBS and 0.5 ng/mL TGF-β. The SSCs were inoculated in the culture plate at 5×105/mL density at 37 °C, 5% CO2.
Specific inhibit Wnt/β-catenin signaling pathways of SSCs
Axin-RNAi was applied to decrease the expression of Axin protein, and specifically inhibit the Wnt/β-catenin signaling pathway of the SSCs. Second to 5th generation SSCs were subcultured in low glucose DMEM with 10% FBS and Axin-RNAi (100 MOI). DKK1 was chose as the positive control. The SSCs were inoculated in the culture plate at 5×105/mL density at 37 °C, 5% CO2.
Induce SSCs to differentiate into fibroblasts combine with specific inhibition of Wnt/β-catenin signaling pathway
SSCs were induced to differentiate into fibroblast by TGF-β. Axin-RNAi was used to inhibit the Wnt/β-catenin signal pathway. Second to 5th generation SSCs were subcultured in low glucose DMEM with 10% FBS and 0.5 ng/mL TGF-β + Axin-RNAi (100 MOI). DKK1 was chose as the positive control. The SSCs were inoculated in the culture plate at 5×105/mL density at 37 °C, 5% CO2.
Cell viability and proliferation detection
Viability and proliferation of SSCs was detected by MTT assay from the 1st to 10th day after treatment. In brief, SSCs cells were cultured in 96-well plates. The cells were incubated with 25 µL MTT solution (5 mg/mL) for 4 hours at room temperature, supernatant was discarded and 100 µL DMSO was added to dissolve the formazan precipitate for half an hour at 37 °C. Optical density was measured at 570 nm with an automatic microplate spectrophotometer (SpectraMax 190, Sunnyvale, USA).
The key protein of Wnt/β-catenin signaling pathways expression
Protein β-catenin, CD14, p-ERK and p-Jun N-terminal kinase (p-JNK) expression in Wnt/β-catenin signaling pathways of SSCs after treatment for each group was detected by cell immunofluorescence. Briefly, a further three generations of SSCs were cultivated according to the grouping method. SSCs were fixed in precool methanol for 20 minutes. Sealed non-specific protein in 1% bovine serum albumin (BSA) for 1 hour. Cells were then incubated in diluting primary antibodies for 2 hours at 37 °C (rabbit anti-mouse β-catenin, CD14, p-ERK and p-JNK) (Abcam Inc., Cambridge, MA), followed by the secondary antibody for 1 hour at 37 °C [fluorescein labeled antibody to rabbit IgG (H + L); 1:100]. The cell nucleus was incubated with diamidino-phenyl-indole for 10 minutes at room temperature. Cells were washed 5 times by PBST between each step. Fluorescence was detected and recorded by the inverted fluorescence microscope.
The expression of fibroblast surface markers in SSCs
The expression of fibroblast surface markers vimentin, α-smooth muscle actin (α-SMA) and the anti-fibroblast antibody, TE-7 in SSCs was analysed by western blotting. Three further generations of SSCs were cultivated according to the grouping method. Then, cells were lysed in ice-cold extraction buffer containing protease inhibitor cocktail (Roche) and centrifuged at 10,000 rpm for 10 minutes. The protein concentration in the supernatant was determined using Bradford assays. Proteins were separated using 4% concentrated gel and 10% sodium dodecyl sulfate polyacrylamide gel electrophoresis and electrophoretically transferred to polyvinylidene fluoride membranes. The membranes were sealed at 37 °C for 1 hour in PBST and 5% (wt/vol) non-fat milk. Membranes were then incubated in primary antibody at a 1:500 dilution in PBSTM for 2 hours at room temperature and the appropriate horseradish peroxidase (HRP)-conjugated anti-species secondary antibodies (Boster, Wuhan, China) for 1 hour at room temperature then washed in PBSTM as described above. Immunoreactive protein bands were detected using an Odyssey Scanning System (LI-COR, Inc., Lincoln, NE, USA). Ratios for the protein of interest (POI) were expressed relative to glyceraldehyde 3-phosphate dehydrogenase in the same sample as a loading control. The primary antibodies rabbit anti-vimentin, α-SMA, TE-7, and glyceraldehyde 3-phosphate dehydrogenase were obtained from Santa Cruz (USA).
mRNA expression of fibroblast markers in SSCs
mRNA expression level of the fibroblast surface markers vimentin, α-SMA and TCF were analysed by real-time reverse transcription polymerase chain reaction (qRT-PCR). Primers and probes are shown in Table 1.
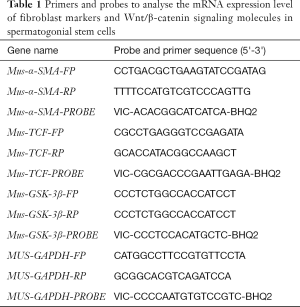
Full table
Statistical analysis
Each experiment was performed in sextuplicate and repeated a minimum of three times. Results are expressed as mean ± SEM. All calculations and statistical analyses were performed using SPSS 18.0. T-tests and one-way ANOVA followed by Tukey honest significance test were used to analyse data differences. P<0.05 was considered statistically significant.
Results
SSCs culture in vitro
The morphology of cultured SSCs was observed by the microscope. Some cells adhered to the bottom of the flasks after 3 days. Seven days later, colonies of SSCs, including single and double spheres, and clusters, grew uniformly into spiral shape or long shuttle type. After 14 days, the cells were a mixed 80% fusion. After 3 generations, the SSCs grew faster, and in a unified form (Figure 1).
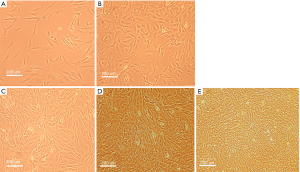
Surface molecular phenotype identify of SSCs by FACS
Flow cytometry analysis showed that the 3rd generation of SSCs expressed CDH1, ID4, NAN0S3 and CD44, but did not express CD31 or CD45 (Figure 2), which was consistent with the characteristics of surface molecular markers of SSCs. The purity of SSCs was approximately 95%. However, for the 10th generation of SSCs, only CD44 was expressed (Figure 3). The experiments were performed in triplicate.
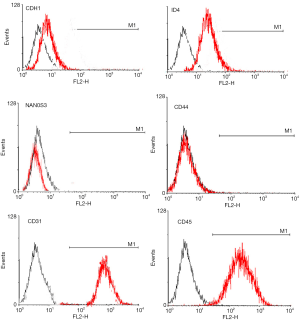
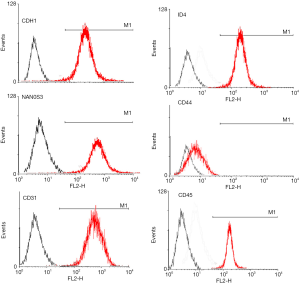
The observation of SSCs cells after transfection
Fluorescence microscopy observation
The Axin-RNAi transfection positivity of SSCs cells was observed by fluorescence microscopy, and the proliferation of SSCs was detected by MTT assay after transfection. We found the transfection positivity of SSCs in the 100 and 200 MOI groups were high than in the 10 MOI group, and no obvious pathological changes were found among the cells in the control, 10 and 100 MOI transfected groups (Figure 4).
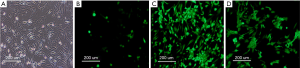
Western blotting analysis
Western blotting results showed that after 48 hours the expression of the Axin protein in the 100 and 200 MOI transfected groups were significantly decreased compared with those in the control and 10 MOI transfected groups (Figure 5).
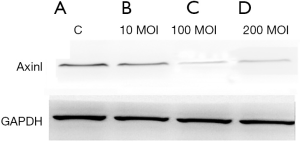
MTT detection
MTT results showed there was no significant difference in the proliferation ability of SSCs in the 10 and 100 MOI Axin-RNAi transfected groups compared with the control group in the 10 days post transfection, while the proliferation ability of SSCs in the 200 MOI transfected group was decreased significantly compared with the control group (Figure 6).
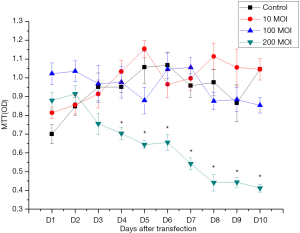
These indicated that 100 MOI Axin-RNAi virus solution transfection could reduce the expression of Axin protein, and had no significant effect on the proliferation ability and cell activity of SSCs cells.
Influence of Lenti-Axin-si transfection on SSCs differentiation
MTT assay was used for detecting the proliferation differences in the different groups of SSCs. It was found that TGF-β could influence the proliferation ability of SSCs. The proliferation ability was significantly promoted in SSCs + TGF-β group, with the obviously random arrangement of cells. However, in SSCs + Axin-RNAi group, proliferation activity of SSCs was retarded, maintaining uniform arrangement, long spindle and swirling cell morphology. There was no significantly difference between the SSCs + Axin-RNAi group and the SSCs + DKK1 group, also shown by MTT test (Figure 7).
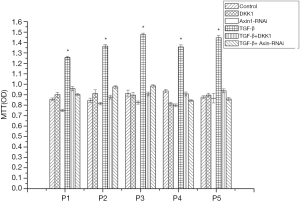
Protein expression level of Wnt/β-catenin signaling pathway in SSCs
Protein β-catenin, CD14, p-ERK and p-JNK expression of Wnt/β-catenin signaling pathways in the different groups of SSCs after treatment were detected by cell immunofluorescence.
When TGF-β was added to the culture, the Wnt/β-catenin signaling pathway was highly activated. In the SSCs + TGF-β group, β-catenin, CD14, p-ERK and p-JNK protein expression increased significantly 3 days later. The expression of these proteins in the SSCs + TGF-β + DKK1 group and the SSCs + Axin-RNAi group were reduced in comparison with MSCs + TGF-β (Figure 8).
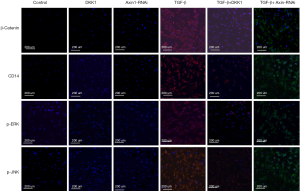
Expression of fibroblast surface markers
The expression of fibroblast surface markers (vimentin, α-smooth muscle actin (α-SMA) and TE-7) in different groups of SSCs were analysed by western blotting. The results showed that the expression levels of vimentin, α-SMA and TE-7 were up-regulated in the SSCs + TGF-β group, while in the SSCs + TGF-β + Axin-RNAi group the expression levels were close to the control group (Figure 9).
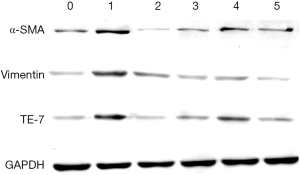
mRNA expression of fibroblast markers in SSCs
mRNA expression level of fibroblast surface markers vimentin, α-SMA and TCF were analysed by qRT-PCR. The results showed that they were significantly increased in MSCs of the SSCs + TGF-β group compared with the SSCs + TGF-β + Axin-RNAi group, in which expression levels of fibroblast surface markers were close to the control group (Figure 10).
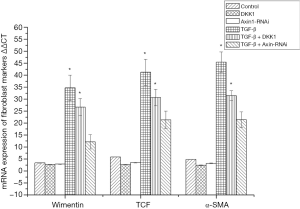
Discussion
Spermatogenesis is a series of complex processes of sequential cell proliferation and differentiation in male testis, affected by many crucial proteins and signal pathways (15,16). Because SSCs produce a large number of progenitors, they are important in the spermatogenesis process (17). In a previous study we found that the use MSCs could lead to a significant increase in the expression of fibroblast growth factor and TGF-β both in serum and testis tissue of mice, which may lead to fibrosis of cells and tissues. Previous studies have shown that the mitogen-activated protein kinases (MAPK) regulates and activates the Wnt signaling pathway, could influencing spermatogonia via the non-canonical Wnt pathway.
The results of this study indicate that TGF-β can activate the Wnt/β-catenin signaling pathway by upgrading the expression of some key proteins in the Wnt signaling pathway. Subsequently, the proliferation of SSCs was significantly increased, but the majority of these cells proved to differentiate into fibroblasts. These findings are similar to research by Yeh et al. which reported that Wnt3a can activate the β-catenin pathway in a subset of cluster cells (18). However, SSCs show a tendency to differentiate under this environment. Our results indicate that the activation of canonical Wnt/β-catenin signal pathway could induce the SSC proliferation in vitro, and SSC might be present a tendency of fibrosis by influencing spermatogonia via the canonical Wnt pathway.
It has been reported that the Wnt signaling pathway, including Wnt ligands, Wnt protein receptors and downstream transcription factors, plays an important role in cell proliferation and differentiation (19,20). Our results showed that TGF-β can activate the Wnt/β-catenin signaling pathway by upgrading the expression of the key proteins β-catenin, CD14, p-ERK and p-JNK, which leads to upgraded expression of the fibroblast surface markers vimentin, α-SMA, TCF and TE-7, which subsequently include differentiation of SSCs into fibroblasts. These results were similar to those in the study by HE, which stated that PKC is specially involved in the Wnt5a-induced differentiation of chicken embryonic stem cells and SSCs (21). Therefore, how to regulate the differentiation direction of SSCs, maintain their normal cell characteristics, and avoid the tendency of fibroblast differentiation, could be a valuable focus for SSCs research.
We speculated that some signals of canonical Wnt pathway might be the key molecules to regulate the differentiation direction of SSCs. In order to confirm this inference, we constructed an interference sequence siRNA of Wnt signaling pathway key molecular Axin gene and transfected it into SSCs, to regulate the activity of Wnt/β-catenin signaling pathway by down-regulate the expression of Axin. Our results proved that once Axin-RNAi was applied, the Wnt signaling pathway in SSCs could be relatively inhibited. Immunofluorescence detection showed that the expression levels of the Wnt signaling pathway molecules such as β-Catenin, TCF, GSK-3β, Axin, and JNK tended to be normal in SSCs. Western blot and real-time PCR were used to detect vimentin, α-SMA, TCF and TE-7. We found that the expression levels of fibroblast markers vimentin, α-SMA, TCF and TE-7 were close to the control group. The canonical Wnt pathway is referred to as the β-catenin pathway. The key effect of this pathway including β-catenin, Axin, and JNK which are commonly found in the cytoplasm of cells. On activation of the canonical Wnt pathway, the activated key protein could regulate the transcription of targeted genes. In our present study we deduced the regulation of canonical Wnt pathway could influence the differentiation direction of SSCs. This study is a mechanism study at the cellular level, and further study on its animal level and human mechanism is needed.
Conclusions
In summary, our study suggests that the Wnt/β-catenin signaling pathway contributes to SSC fibroblast differentiation. In addition, we showed that an Axin-RNAi interference virus could block Wnt/β-catenin signal pathway activation by inhibiting Axin protein expression. Specific inhibition of the Wnt/β-catenin signaling pathway can inhibit the differentiation of SSCs into fibroblasts and be valuable for clinical application of SSCs.
Acknowledgments
Funding: None.
Footnote
Reporting Checklist: The authors have completed the ARRIVE reporting checklist. Available at http://dx.doi.org/10.21037/atm-20-5321
Data Sharing Statement: Available at http://dx.doi.org/10.21037/atm-20-5321
Conflicts of Interest: All authors have completed the ICMJE uniform disclosure form (available at http://dx.doi.org/10.21037/atm-20-5321). The authors have no conflicts of interest to declare.
Ethical Statement: The authors are accountable for all aspects of the work in ensuring that questions related to the accuracy or integrity of any part of the work are appropriately investigated and resolved. All animal experiments were performed in accordance with the guidelines for animal care and the protocol was approved by the Committee on the Ethics of Animal Experiments of Jiangsu Provincial Center for Disease Prevention and Control, (approval no. JSJK/JL-161).
Open Access Statement: This is an Open Access article distributed in accordance with the Creative Commons Attribution-NonCommercial-NoDerivs 4.0 International License (CC BY-NC-ND 4.0), which permits the non-commercial replication and distribution of the article with the strict proviso that no changes or edits are made and the original work is properly cited (including links to both the formal publication through the relevant DOI and the license). See: https://creativecommons.org/licenses/by-nc-nd/4.0/.
References
- Yamasaki S, Yoshida S, Kato K, et al. Effects of stem cell transplantation in patients with peripheral T-cell lymphoma not otherwise specified and angioimmunoblastic T-cell lymphoma. Int J Hematol 2020;112:74-83. [Crossref] [PubMed]
- Mohseni M, Shojaei S, Mehravi B, et al. Natural polymeric nanoparticles as a non-invasive probe for mesenchymal stem cell labelling. Artif Cells Nanomed Biotechnol 2020;48:770-6. [Crossref] [PubMed]
- Sharma A, Kumaresan A, Mehta P, et al. Successful transplantation of transfected enriched buffalo (Bubalus bubalis) spermatogonial stem cells to homologous recipients. Theriogenology 2020;142:441-9. [Crossref] [PubMed]
- Zhao H, Li T, Yang H, et al. The effects of growth factors on proliferation of spermatogonial stem cells from Guangxi Bama mini-pig. Reprod Domest Anim 2019;54:1574-82. [Crossref] [PubMed]
- Kanatsu-Shinohara M, Yamamoto T, Toh H, et al. Aging of spermatogonial stem cells by Jnk-mediated glycolysis activation. Proc Natl Acad Sci U S A 2019;116:16404-9. [Crossref] [PubMed]
- Paucarmayta A, Taitz H, Casablanca Y, et al. TGF-β signaling proteins and CYP24A1 may serve as surrogate markers for progesterone calcitriol treatment in ovarian and endometrial cancers of different histological types. Transl Cancer Res 2019;8:1423-37. [Crossref]
- Schmitt M, Schewe M, Sacchetti A, et al. Paneth Cells Respond to Inflammation and Contribute to Tissue Regeneration by Acquiring Stem-like Features through SCF/c-Kit Signaling. Cell Rep 2018;24:2312-28.e7. [Crossref] [PubMed]
- Yu S, Cao S, Hong S, et al. miR-3619-3p promotes papillary thyroid carcinoma progression via Wnt/β-catenin pathway. Ann Transl Med 2019;7:643. [Crossref] [PubMed]
- Golestaneh N, Beauchamp E, Fallen S, et al. Wnt signaling promotes proliferation and stemness regulation of spermatogonial stem/progenitor cells. Reproduction 2009;138:151-62. [Crossref] [PubMed]
- ShamsEldeen AM, Ashour H, Shoukry HS, et al. Combined treatment with systemic resveratrol and resveratrol preconditioned mesenchymal stem cells, maximizes antifibrotic action in diabetic cardiomyopathy. J Cell Physiol 2019;234:10942-63. [Crossref] [PubMed]
- Gu Q, Tian H, Zhang K, et al. Wnt5a/FZD4 Mediates the Mechanical Stretch-Induced Osteogenic Differentiation of Bone Mesenchymal Stem Cells. Cell Physiol Biochem 2018;48:215-26. [Crossref] [PubMed]
- Ibtisham F, Honaramooz A. Spermatogonial stem cells for in vitro spermatogenesis and in vivo restoration of fertility. Cells 2020;9:745. [Crossref] [PubMed]
- Cai Y, Wang J, Zou K. The Progresses of spermatogonial stem cells sorting using fluorescence-activated cell sorting. Stem Cell Rev Rep 2020;16:94-102. [Crossref] [PubMed]
- Ko K, Arauzo-Bravo MJ, Kim J, et al. Conversion of adult mouse unipotent germline stem cells into pluripotent stem cells. Nat Protoc 2010;5:921-8. [Crossref] [PubMed]
- Sujit KM, Singh V, Trivedi S, et al. Increased DNA methylation in the spermatogenesis-associated (SPATA) genes correlates with infertility. Andrology 2020;8:602-9. [Crossref] [PubMed]
- Zhao X, Yang HQ. (Progress on spermatogonial stem cells of large animals. Yi Chuan 2019;41:686-702. [PubMed]
- Valdivia M, Castaneda-Zegarra S, Levano G, et al. Spermatogonial stem cells identified by molecular expression of PLZF, integrin beta1 and reactivity to Dolichos biflorus agglutinin in alpaca adult testes. Andrologia 2019;51:e13283. [Crossref] [PubMed]
- Yeh JR, Zhang X, Nagano MC. Indirect effects of Wnt3a/beta-catenin signalling support mouse spermatogonial stem cells in vitro. PLoS One 2012;7:e40002. [Crossref] [PubMed]
- Jin L, Cao Y, Yu G, et al. SFRP2 enhances the osteogenic differentiation of apical papilla stem cells by antagonizing the canonical WNT pathway. Cell Mol Biol Lett 2017;22:14. [Crossref] [PubMed]
- Yu WZ, Chen XM, Niu WB, et al. Role of Wnt5a in the differentiation of human embryonic stem cells into endometrium-like cells. Int J Clin Exp Pathol 2015;8:5478-84. [PubMed]
- He N, Wang Y, Zhang C, et al. Wnt signaling pathway regulates differentiation of chicken embryonic stem cells into spermatogonial stem cells via Wnt5a. J Cell Biochem 2018;119:1689-701. [Crossref] [PubMed]
(English Language Editor: J. Brown)