Assessing breathing effort in mechanical ventilation: physiology and clinical implications
Introduction
In the past decade, multiple important studies have underlined that maintaining patient breathing effort during mechanical ventilation is a double-edged sword (1-5). Positive effects of patient breathing effort may include improved recruitment of basal lung fields and facilitated oxygenation (5,6). Furthermore, preserving patient breathing effort might protect against development of diaphragm atrophy and contractile dysfunction resulting from disuse (2). On the other hand, patients with high respiratory drive can generate pressures that are incompatible with lung protective-ventilation, a phenomenon termed patient self-inflicted lung injury (P-SILI) (7). Additionally, studies in the early course of acute respiratory distress syndrome (ARDS) have demonstrated that continuous infusion of the neuromuscular blocker cisatracurium improves survival, possibly by abolishing breathing effort (4). Striking a balance between the beneficial and detrimental effects of breathing effort is one of the contemporary challenges in mechanical ventilation management (8). It has been proposed that ventilator assist should be titrated to the individual patient’s disease state, based on the respiratory drive, pressure output of the muscles and lung mechanics (5,8-10).
However, it is difficult to assess activity of the respiratory muscle pump during mechanical ventilation without specific diagnostic techniques (11). The “gold standard” parameters are the work of breathing (WOB) and pressure-time product (PTP), which are based on pressure measurements (12). These measurements can be difficult to obtain and interpret. As such, the PTP and WOB are rarely used in clinical care and are mostly considered to be a research tool (13). Recently, diaphragm electromyography (9) and ultrasound (14) have become increasingly popular to assess breathing effort in research and clinical care. The aim of this review is to describe the physiological basis of breathing effort assessment. We will discuss how esophageal pressure (Pes), gastric pressure (Pga), PTP, WOB, ultrasound and electromyography can be used to quantify breathing effort during mechanical ventilation, and highlight the technical issues related to these measurements. Furthermore, we will discuss which levels of breathing effort may be considered desirable during mechanical ventilation at different stages of critical illness.
Physiology
Definition of breathing effort
Although the term breathing effort feels intuitive there is no clear definition, and many authors and textbooks define it differently. In this review we have defined breathing effort as any energy-consuming activity of the respiratory muscles aimed at driving respiration.
Function of the respiratory muscle pump
The respiratory muscle pump compromises multiple skeletal muscles that act in a coordinated fashion to maintain alveolar ventilation under different metabolic demands. Breathing effort is tightly controlled to match the respiratory demands of the body. An imbalance between breathing effort and the respiratory demands of the body will result in respiratory failure. Mechanical ventilation is life-saving in respiratory failure by taking over the patient’s WOB, restoring the balance between respiratory load and capacity. During partially supported ventilation, the WOB is shared by the patient and the ventilator. To assess the patient’s relative contribution to ventilation, it is useful to separate the inspiratory and expiratory muscle pump. See Figure 1 and Table 1 for a schematic representation of the respiratory system, muscle pressures and pressure gradients.
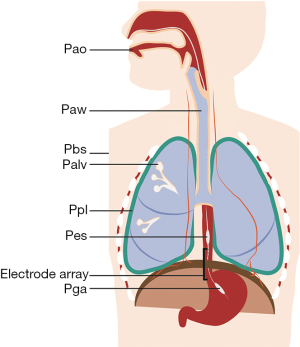
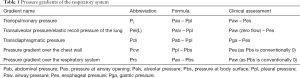
Full table
Inspiratory muscle pump
Inspiration is mainly driven by the diaphragm in healthy individuals during tidal breathing (15). The diaphragm is a thin (±2.0 mm) dome-shaped muscle that forms the boundary between the thorax and the abdomen (16). The muscle fibers are conventionally divided into two main components: the crural portion inserts into the first three lumbar vertebrae, and the costal portion projects onto the rib cage and xiphoid process. The muscle fibers of the costal diaphragm that directly appose to the lower rib cage constitute the “zone of apposition” (16-18). In simplified form, the diaphragm acts like a piston in a barrel. Shortening of the muscle fibers in the zone of apposition descends the dome of the diaphragm, increasing the size of the thoracic cavity and compressing the abdominal cavity. Consequently, intrapleural pressure (Ppl) falls and abdominal pressure (Pab) rises, creating a pressure gradient called the transdiaphragmatic pressure (Pdi) (19,20).
Pdi (cmH2O) = Pab (cmH2O) – Ppl (cmH2O) [1]
The drop in pleural pressure will generate a pressure gradient over the lungs, the transpulmonary pressure (PL), which can be calculated by subtracting Ppl from the airway opening pressure (Pao):
PL (cmH2O) = Pao (cmH2O) – Ppl (cmH2O) [2]
The cyclic rises and falls in PL ultimately drive alveolar ventilation.
Clinically, Ppl and Pab are often estimated by Pes and Pga. The assessment of Ppl and Pab requires placement of esophageal and/or gastric balloons (Figure 1), which may be perceived as too invasive for some patients. Today, balloon catheters are available that can be used for gastric feeding as well and thus assessing Pes and/or Pga is not more invasive than routine clinical care (13). It is important to note that the balloon catheters provide an estimation of pleural pressure, but the actual pressure in the pleural space differs from region to region due to gravity and differences in spatial respiratory mechanics (21). Nevertheless, Pes measurements provide a useful estimation of the mean pleural pressure at the dependent lung regions (21,22). The advantages, limitations and technical aspects of Pes and Pga measurements in critically ill patients have been discussed in two excellent review articles (23,24).
Additional muscle groups are recruited when the respiratory load is elevated. The most important accessory inspiratory muscles are the sternomastoid, parasternal, scalene and rib cage muscles (25). Like the diaphragm, contraction of the accessory inspiratory muscles expands the thorax and lowers Ppl, promoting a rise in PL and subsequent lung inflation. Contraction of accessory inspiratory muscles does not generate a pressure gradient between the abdominal and thoracic compartment if the diaphragm is relaxed (12). At any time, Ppl depends on the pressure generated by all the respiratory muscles (Pmus) and the pressure gradient over the chest wall (Pcw):
Ppl (cmH2O) = Pmus (cmH2O) + Pcw (cmH2O) [3]
Accordingly,
Pmus (cmH2O) = Ppl (cmH2O) – Pcw (cmH2O) [4]
Pmus provides a global assessment of all inspiratory muscles, while Pdi is specific to the diaphragm (12,26). Pcw is often calculated by dividing the inspired volume by the theoretical compliance of the chest wall (Ccw), estimated as 4% of vital capacity. Accordingly, a (predicted) vital capacity of 4,000 mL will reveal an estimated Ccw of 160 mL/cmH2O (12). Reference values for Ccw are between 150 and 200 mL/cmH2O (12). However, these values were obtained in healthy subjects and might not be accurate in critical illness. The actual Ccw of a patient can be determined by constructing a pressure-volume curve for Pes during passive inflation; Pmus is zero during passive inflation and muscle paralysis, meaning the observed Pes is completely determined by Pcw {Eq. [3]} (26).
Expiratory muscle pump
Expiration is a passive process during quiet breathing (19,26,27). When the inspiratory muscles relax, the elastic energy build up in the structures of the respiratory system drives lung deflation. The expiratory muscles are recruited to assist in expiration when the load posed on the inspiratory muscles is elevated (28). Additionally, the expiratory muscles are recruited when passive expiration is hampered by reduced elasticity of the lungs (e.g., emphysema) or elevated expiratory resistance [e.g., exacerbation of chronic obstructive pulmonary disease (COPD)] (29). The abdominal wall muscles are the principal muscles of expiration. The internal interosseous intercostal and the triangularis sterni muscles are accessory expiratory muscles (25). Contraction of the abdominal wall muscles compresses the abdominal compartment, increasing Pab. If the diaphragm is relaxed, the increased Pab will be transmitted to the thorax and increase Ppl, facilitating lung deflation. Contraction of the accessory expiratory muscles directly increases Ppl by compressing the thorax. Notably, the expiratory muscles can also facilitate inspiration. By contracting during the expiratory phase, lung volume is reduced below functional residual capacity and the diaphragm is shifted cephalad to a more optimal position (30,31). When the expiratory muscles relax during the subsequent inspiration, the diaphragm will descend and Ppl will fall, facilitating lung inflation (30). During high respiratory loads the expiratory muscles might generate more pressure than the diaphragm (32). Therefore, assessment of breathing effort at high loads should also take the expiratory muscles into account.
Quantifying breathing effort
Physical examination and graphical inspection of ventilator waveforms
Physicians may rely on physical examination to assess breathing effort in clinical practice. For instance, recruitment of accessory muscles is a sign of increased respiratory workload (32). Inward movement of the abdomen during inspiration (abdominal paradox) means the accessory muscles exert more force than the diaphragm, which is often interpreted as a sign of impending diaphragm fatigue (33). Most patients develop a breathing pattern characterized by low tidal volumes and high respiratory frequency during prolonged fatiguing loads (34). However, these breathing patterns suggest an increased workload and impending fatigue, but do not allow quantitative assessment of breathing effort (35). The pressure and flow waveforms displayed on the ventilator are also inadequate in assessing breathing effort during partially supported ventilation, as they cannot distinguish between patient breathing effort and work of the ventilator (11).
Pressure-based quantification of breathing effort
Because the respiratory muscles exert their function by generating pressure, breathing effort can be assessed by analysis of these pressures. The requirements and reference values of pressure-based assessment of breathing effort are summarized in Table 2.
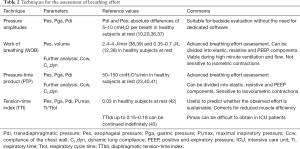
Full table
Pressure amplitudes
If an esophageal and a gastric balloon are present, the amplitude of tidal swings in Pes, Pdi and Pga can be studied during partially supported ventilation by using the following calculation:
ΔP (cmH2O) = P, expiration (cmH2O) − P, inspiration (cmH2O) [5]
The formula can be used with Pes, Pga and Pdi to obtain their respective amplitude swings during inspiration and expiration. If the patient’s respiratory muscles are active, Pes will fall and Pga and Pdi will rise during inspiration (Figure 2A,B,C,D). A rise in Pga during expiration is a sign of expiratory muscle recruitment.
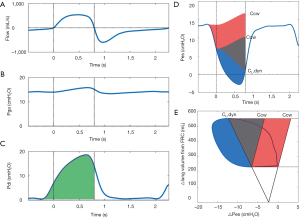
Pressure amplitude assessment is relatively straightforward and can be performed real-time at the bedside, making it especially useful to observe trends within a patient. However, there are several limitations to assessment of breathing effort based solely on Pes and Pga swings. Amplitude of pressure swings neglects the duration and frequency of contractions. Additionally, Pes swings are usually not corrected for the recoil pressure of the chest wall and intrinsic positive end-expiratory pressure (PEEPi), which can result in both under- and overestimation of Pmus. Consequently, pressure measurements show a rather poor correlation to energy expenditure (35). More in-depth analysis of breathing effort may be warranted in selected critically ill patients.
PTP
A more sophisticated parameter to quantify breathing effort is the PTP. The PTP is calculated as the time-integral of the Pmus (12):
PTP = P (cmH2O) × t(s) = ∫ Pdt (cmH2O*s) [6]
PTP is commonly reported over a 1-minute interval. The PTP of the respiratory muscle pressure (PTPmus) can be constructed if Pes measurements are available as an assessment of global respiratory muscle activity (Figure 2D). If Pga is also available, the PTP of the diaphragm pressure (PTPdi) can be constructed as a specific measurement of diaphragm effort (Figure 2C). Because the PTP is sensitive to the frequency and duration of contractions it correlates well with energy expenditure during a broad range of inspiratory loads (44). The PTP is insensitive to changes in volume, meaning that it is also valid when effort does not result in volume generation, such as during isometric contractions. This is especially relevant in ICU patients with PEEPi and poor interaction with the ventilator, that exhibit ineffective efforts (45). The PTP of the esophageal pressure (PTPes) can be divided into parts to overcome elastic, resistive and threshold (i.e., PEEPi) forces (Figure 2D). This subdivision may be of clinical interest in patients difficult to wean the ventilator, or to monitor effects of ventilator management and pharmacological interventions. The technical aspects of measuring the PTP have been covered recently (23,24). It is also possible to construct a PTP of the expiratory muscles (PTPex) (46), but this technique is seldom used and reference values are not available. More research is required before overall recommendations on PTPex can be made.
There are limitations to the PTP. Volume and flow are not considered, even though contractions at higher flows and volumes are less efficient and thus require more effort. This has been demonstrated in studies where equal PTPs were generated at different flows and volumes. This resulted in widely different levels of the oxygen cost of breathing at equal PTPs (44,47). Furthermore, calculation of PTPmus requires measurement of Ccw, which is cumbersome in patient on partially supported ventilation as it requires passive inflation and muscle paralysis (12). Despite these limitations, the PTP is very useful as it is linearly related to activity and energy expenditure of the respiratory muscle pump during relatively constant ventilation. This was demonstrated in conditions of flow below 1 L/s (48) and duty cycles between 0.3 and 0.6 (44), which is applicable to most ICU patients (36).
Tension-time index (TTI)
Another method to assess breathing effort is the TTI, which relates the average inspiratory pressure (Pi,mean) to the maximal inspiratory pressure (Pi,max) that a patient can generate:
TTI = (Pi,mean/Pi,max)*(Ti/Ttot) [7]
In which Pi,mean can be either Pdi,mean or Pmus,mean, and Ti/Ttot is the relative duration of inspiration to a full breath cycle. For example, generating a Pdi,mean equal to 30% of Pi,max at a duty cycle of 0.5 would yield a diaphragmatic TTI (TTIdi) of 0.15. Some ventilators can calculate Pi,mean over a time period of a few breaths. Additionally, it is possible to obtain the Pi,mean by dividing the inspiratory PTP by the sampling period (12). The advantage of TTI over other indices is that the TTI partially corrects for reductions in muscle efficiency and weakness by relating the observed pressures to the maximal pressures. The TTI correlates well with oxygen consumption of the respiratory muscle pump (43,44). The TTI is also correlated with the time a certain load can be upheld by the diaphragm. In healthy individuals a TTIdi below 0.15–0.18 can be sustained indefinitely, while higher values will eventually lead to fatigue and task failure (43,49).
There are some technical and theoretical limitations of the TTI. Technically, it is difficult to obtain reliable Pi,max-measurements in critically ill patients as it requires maximal voluntary effort which is hindered by sedation and motivation (12). Furthermore, the TTI does not take volume and flow into account. This was illustrated in two studies where the maximal sustainable TTIdi ranged from 0.11 to 0.22 and maximal sustainable TTI of the respiratory muscles (TTImusc) ranged from 0.16 to 0.32 within the same subject, depending on flow, volume and duty cycles (50,51). Despite these limitations, the TTI is a clinically useful parameter especially to assess whether a load posed on the patient’s respiratory muscle pump is sustainable.
WOB
The classic method to assess breathing effort is the WOB. Work is done when a force moves its point of application over a distance. In case of the respiratory system work is done when a pressure changes the volume of the system (26).
WOB = P (cmH2O) × V (L) = ∫ P dv (J) [8]
WOB is often reported as work per liter (J/L), obtained by dividing the work per breathing cycle by the tidal volume. Increased work per liter means that more pressure is required to generate an equal volume. This can be caused by several factors, such as reduced lung compliance or the presence of PEEPi (52). Detailed analysis of the WOB is possible using the Campbell diagram to divide work into resistive, elastic and PEEPi components (Figure 2E) (23,26,52). Furthermore, WOB of the expiratory muscles can be assessed by attributing any observed Pes above the Ccw curve to expiratory muscle activity. Additionally, work per breathing cycle can be multiplied by the respiratory rate (in breaths per minute) to obtain the power of breathing or work rate (12). This is an interesting parameter from a physiological point of view, as it combines time and volume dimensions. Work rate correlates closely to oxygen consumption of the respiratory muscles in a wide range of flows, volumes and duty cycles (44,47). It has been proposed that the work rate, or mechanical power, is a unifying factor that might predict development of ventilator-induced lung injury (VILI) (53,54).
There are limitations to WOB. First, as work is only performed when a volume is displaced, the WOB is insensitive to isometric contractions. Second, duration and frequency of contractions are also not considered. For example, the same breath might generate the same tidal volume at the same Pes, but could take twice as long. Work would not be different between these two breaths, even though the longer breath will consume substantially more energy.
Other methods to quantify breathing effort
Other techniques to quantify breathing effort that do not depend on direct assessment of pressure have been developed, including the electrical activity of the diaphragm (EAdi) and ultrasound. See Tables 3,4 for details, including reference values on these techniques.
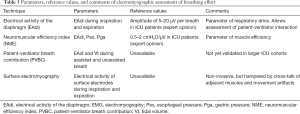
Full table
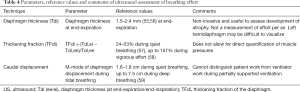
Full table
Respiratory muscle electromyography
Neural control of breathing effort is tightly matched to the respiratory demands of the body. As such, both surface electrodes and electrodes placed on a nasogastric tube have been used to acquire electromyographic signals of the respiratory muscles in order to assess breathing effort in research and clinical care.
Surface electrodes have been used to measure activity of the diaphragm, accessory respiratory muscles and expiratory muscles. Although non-invasive, the quality of the recordings can be heavily impaired by cross-talk of adjacent muscles and other factors such as fat, edema and movement artifacts (60,61). Furthermore, there are no standardized procedures for placement and analysis of the surface electrode signals and no reliable reference values are available. Therefore, further study is required before this technique can be used to quantify breathing effort in clinical care.
The EAdi-signal circumvents some of the technical difficulties of surface electromyography. The EAdi-signal can be monitored real-time using a dedicated naso-gastric tube with wired electrodes positioned at the level of the crural diaphragm (Figure 1) (62). This catheter was originally designed to control the ventilator in a specific ventilation mode [neurally adjusted ventilatory assist (NAVA)], but recent reports indicate that EAdi-recordings are useful to monitor breathing effort and patient-ventilator interaction (63-65). Measurement of EAdi does generally not require additional invasive procedures as most ventilated ICU patients are instrumented with a feeding tube for regular care. The electrodes acquire the spatial and temporal summation of action potentials from the motor units in the crural diaphragm. There is a close correlation between electrical activity from the crural and costal parts of the diaphragm (66). Furthermore, the EAdi-signal is independent of changes in lung volume (67). EAdi correlates well to Pdi in healthy individuals and ICU patients (67). Thus, EAdi appears to be a reliable estimate of global diaphragm muscle activity in ICU patients (68-70).
Electrical activity is not synonymous with muscle contraction and force generation. The coupling between electrical activity and pressure is expressed as the neuromuscular efficiency index (NME):
NME (cmH2O/µV) = Pdi (cmH2O)/EAdi (µV) [9]
The NME can be used to calculate pressure from EAdi when assuming constant coupling over time. NME obtained during expiratory holds and multiplied by the observed EAdi appeared to be a reliable estimation of Pmus under different conditions of ventilator assistance (9). Another index derived from the EAdi is the patient-ventilator breath contribution (PVBC). The PVBC estimates the patient’s relative contribution to tidal volume generation during NAVA by comparing EAdi peaks with tidal volume in assisted and non-assisted breaths (71). The PVBC reliably predicted the fraction of breathing effort generated by the patient in a small group of ARDS patients (72).
Although promising, there are still limitations of EAdi-derived parameters to assess breathing effort. The EAdi-derived parameters are not necessarily a direct measure of breathing effort, but are more closely related to neural drive. EAdi is insensitive to recruitment of accessory muscles, making it less suited to assess breathing effort at high workloads. Furthermore, reference values for EAdi-derived parameters are not yet known. NME and PVBC indices need to be further evaluated in larger ICU populations before both indices can be widely implemented in daily clinical practice.
Ultrasound
Ultrasound has gained in popularity as a diagnostic tool in clinical management and research in the ICU (14). The role of ultrasound to evaluate respiratory muscle function and effort have been discussed in recent articles (65,73). Components of the respiratory muscle pump, including the diaphragm, abdominal wall muscles and accessory muscles, are positioned relatively superficial and are readily accessible for ultrasound. Changes in the absolute thickness of the respiratory muscles over time within a patient can be used to recognize the development of atrophy (2,74,75). The thickening fraction of the diaphragm (TFdi) in the zone of apposition during inspiration can be used as a measure of contractile activity (76,77). This requires measurement of diaphragm thickness (Tdi) at end-expiration (Tdi,ee) and end-inspiration (Tdi,ei) (73):
TFdi = (Tdi,ei − Tdi,ee)/Tdi,ee*100% [10]
TFdi has shown fair correlation to the Pdi (78), PTPdi and PTPes (76,77) in some studies, but was not significantly correlated to Pdi in another study (55). Movement of the diaphragm dome during inspiration has also been used to evaluate diaphragm function and reference values are available (Table 4). To study diaphragm movement, the ultrasound probe is placed at the subcostal position, using the liver as a window on the right side and the spleen on the left side. In contrast to the TFdi, assessment of diaphragm movement should only be conducted in patients disconnected from the ventilator, as ventilator assistance will result in caudal movement of the diaphragm, even in a patient on neuromuscular blockers. As such, there is a poor correlation between diaphragm excursion and TFdi, PTPes and PTPdi during partially supported ventilation (77).
Advantages of ultrasound include the non-invasive nature, low-costs, steep learning curve and straightforward calculations which allows bedside evaluation of breathing effort (73). However, several technical and methodological limitations apply to ultrasound assessment of breathing effort. Because the diaphragm is very thin, small errors in measurement can result in large overestimation and underestimation of thickness and thickening fraction (73). Additionally, the left hemidiaphragm is harder to visualize than the right side (78). Furthermore, TFdi is insensitive to duration and frequency of contractions and does not account for recruitment of accessory and expiratory muscles (59). Despite these limitations, ultrasound is a very promising technique in clinical care, especially as a bedside evaluation tool.
Clinical implications
Although assessment of breathing effort has been applied in physiological research and clinical studies for decades, the optimal range of breathing effort in critically ill patients remains to be established. Trials that compare different levels of effort have not been published so far. Therefore, we are dependent on physiological principles and reasoning to guide lung-protective and diaphragm-protective ventilation (79).
Insufficient breathing effort
Studies in the past decade have promoted the idea that insufficient breathing effort leads to atrophy and weakness of the diaphragm, a process termed ventilator-induced diaphragm dysfunction (VIDD) (80,81). For instance, significant diaphragm atrophy has been observed after complete inactivity of the diaphragm for 18 to 69 hours in brain-dead organ donors (1). Subsequent studies demonstrated that diaphragm atrophy also occurs during partially supported ventilation (82-85), and that the extent of atrophy is related to the level of assistance provided by the ventilator (2). Recently, development of diaphragm atrophy has been associated with prolonged ICU admission and an increased risk of complications (86), further supporting the idea of ventilator over-assistance and VIDD.
Additional factors can play a role in the development of muscle weakness and atrophy in ICU patients including inflammation (87), myotoxic drugs (88), nutritional deficiency and catabolic state (89). The term critical illness-associated diaphragm weakness (CIADW) is now preferred over VIDD to describe respiratory muscle weakness in critically ill patients (90). Remarkably, clinical studies have shown that neuromuscular blockers administered in the first 48 hours of moderate to severe ARDS improves outcome without the development of clinically relevant muscle weakness (4,91-93). Recently, it was observed that patients on assist-control ventilation regularly exhibited contractions of the diaphragm, a type of asynchrony called reversed triggering (94). This mechanism prevents complete inactivity of the diaphragm, and possibly hampers the development of disuse atrophy during controlled ventilation. Indeed, short daily sessions of low-frequency pacing prevents development of disuse atrophy in peripheral skeletal muscles of ICU patients (95,96). In a case study on a single patient receiving controlled ventilation for eight months, pacing one hemidiaphragm 30 minutes a day prevented the development of disuse atrophy (97). Although further study is warranted, it is possible that levels below resting breathing effort can prevent development of disuse atrophy (40).
Excessive breathing effort
Prevention of excessive breathing effort by unloading the respiratory muscles is a cornerstone of mechanical ventilation. Excessive effort can be detrimental to lung mechanics and the function of the respiratory muscle pump.
Detrimental effects on the lungs
Vigorous efforts can generate substantial negative pleural pressures (98), potentially leading to injurious PL in ventilated patients (7,99-101). As such, it is advised to keep the peak PL below 25 cmH2O and tidal amplitude swings below 12 cmH2O based on the physiological principles of stress and strain (23,102). Furthermore, vigorous patient effort could result in poor synchrony with the ventilator and impairs oxygenation and comfort (103), warranting sedation and paralysis (91). Reduced Ppl can cause intrapulmonary air shifts from non-dependent to dependent regions (pendelluft), potentially leading to injurious alveolar over-distention and rupture (3). Additionally, the reduced Ppl brought on by vigorous patient effort increases the vascular transmural pressure and can lead to elevated lung perfusion and development of alveolar edema (22,23). Increased activity of the expiratory muscles could result in elevated Ppl during expiration. If the Ppl is higher than alveolar pressure (Palv) the alveoli have a tendency to collapse, promoting atelectasis and possibly cyclic recruitment of alveoli (atelectrauma) (99,104). It is possible that the beneficial effects of muscle relaxants during the early course of ARDS can be attributed to the prevention of excessive muscle effort (4,92,93), although effort was not measured in these studies.
Detrimental effects on respiratory muscles
Excessive breathing effort could incite eccentric contractions of antagonizing muscle groups, for example concomitant activation of the diaphragm and abdominal muscles. Eccentric contractions were found to cause sarcolemmal disruption and inflammation on a microscopic level in animal models (105,106). Furthermore, sarcolemmal disruption has been observed in animal models of mechanical ventilation after high breathing effort (107-109) and in patients with COPD (110). Infiltration of inflammatory cells has been observed in diaphragm fibers obtained from ventilated ICU patients (83). Additionally, patients exhibiting high efforts as assessed by diaphragm ultrasound showed increased Tdi over time, which could be a sign of muscle inflammation and/or injury (2). However, both studies were observational, so whether vigorous breathing effort leads to sarcolemmal damage in critically ill patients, and at which levels of effort this occurs, warrants further study.
Appropriate effort during different stages of illness
Based on the aforementioned considerations we recommend monitoring of breathing effort in selected mechanically ventilated patients (65,111). During the very early course of critical illness respiratory drive can be excessive, leading to injurious respiratory muscle contractions and damaging PL, especially in ARDS (98). In this early phase, high effort occurs in a “muscle hostile environment” characterized by systemic and local inflammation. It is reasonable to prioritize unloading of the respiratory muscles to prevent the lung injury and diaphragm dysfunction under these conditions (7,79). Employing a partially supported mode to regain patient breathing activity is advisable after the initial phase of critical illness, although strenuous efforts should still be prevented (79,101). This strategy has the potential to allow simultaneous lung-protective and diaphragm-protective ventilation.
Conclusions
Both ventilator over-assistance and under-assistance may have adverse effects on respiratory muscle function. As outlined in this review the desired level of respiratory muscle effort depends on patient characteristics, in particular the phase of critical illness and mechanical output of the respiratory muscles. Quantification of breathing effort requires specific monitoring techniques and calculations. This is a developing field, and new studies will help us to better define optimal levels of respiratory muscle activity in ICU patients. The fact that we do not have clinical studies to demonstrate that monitoring of respiratory muscle function improves outcome should not be used as a reason to withhold such techniques in selected patients. Future clinical trials will provide data if guiding ventilator management on breathing effort improves outcome of critically ill patients.
Acknowledgements
H de Vries is supported by a research grant of Amsterdam Cardiovascular Sciences.
Footnote
Conflicts of Interest: L Heunks has received research support from Liberate Medical (USA) and Orion Pharma (Finland), and speakers fee from Getinge (Sweden). The other authors have no conflicts of interest to declare.
References
- Levine S, Nguyen T, Taylor N, et al. Rapid disuse atrophy of diaphragm fibers in mechanically ventilated humans. N Engl J Med 2008;358:1327-35. [Crossref] [PubMed]
- Goligher EC, Fan E, Herridge MS, et al. Evolution of diaphragm thickness during mechanical ventilation:Impact of inspiratory effort. Am J Respir Crit Care Med 2015;192:1080-8. [Crossref] [PubMed]
- Yoshida T, Uchiyama A, Fujino Y. The role of spontaneous effort during mechanical ventilation:Normal lung versus injured lung. J Intensive Care 2015;3:18. [Crossref] [PubMed]
- Papazian L, Forel JM, Gacouin A, et al. Neuromuscular Blockers in Early Acute Respiratory Distress Syndrome. N Engl J Med 2010;363:1107-16. [Crossref] [PubMed]
- Yoshida T, Fujino Y, Amato MBP, et al. Fifty Years of Research in ARDS. Spontaneous Breathing during Mechanical Ventilation. Risks, Mechanisms, and Management. Am J Respir Crit Care Med 2017;195:985-92. [Crossref] [PubMed]
- Putensen C, Zech S, Wrigge H, et al. Long-term effects of spontaneous breathing during ventilatory support in patients with acute lung injury. Am J Respir Crit Care Med 2001;164:43-9. [Crossref] [PubMed]
- Brochard L, Slutsky A, Pesenti A. Mechanical ventilation to minimize progression of lung injury in acute respiratory failure. Am J Respir Crit Care Med 2017;195:438-42. [Crossref] [PubMed]
- Goligher EC, Ferguson ND, Brochard LJ. Clinical challenges in mechanical ventilation. Lancet 2016;387:1856-66. [Crossref] [PubMed]
- Bellani G, Mauri T, Coppadoro A, et al. Estimation of Patient’s Inspiratory Effort From the Electrical Activity of the Diaphragm*. Crit Care Med 2013;41:1483-91. [Crossref] [PubMed]
- Carteaux G, Mancebo J, Mercat A, et al. Bedside Adjustment of Proportional Assist Ventilation to Target a Predefined Range of Respiratory Effort*. Crit Care Med 2013;41:2125-32. [Crossref] [PubMed]
- Colombo D, Cammarota G, Alemani M, et al. Efficacy of ventilator waveforms observation in detecting patient-ventilator asynchrony*. Crit Care Med 2011;39:2452-7. [Crossref] [PubMed]
- American Thoracic Society/European Respiratory Society. ATS/ERS Statement on respiratory muscle testing. Am J Respir Crit Care Med 2002;166:518-624. [Crossref] [PubMed]
- Bellani G, Laffey JG, Pham T, et al. Epidemiology, Patterns of Care, and Mortality for Patients With Acute Respiratory Distress Syndrome in Intensive Care Units in 50 Countries. JAMA 2016;315:788-800. [Crossref] [PubMed]
- Zambon M, Greco M, Bocchino S, et al. Assessment of diaphragmatic dysfunction in the critically ill patient with ultrasound:a systematic review. Intensive Care Med 2017;43:29-38. [Crossref] [PubMed]
- Grimby G, Goldman M, Mead J. Respiratory muscle action inferred from rib cage and abdominal V-P partitioning. J Appl Physiol 1976;41:739-51. [Crossref] [PubMed]
- Troyer AD, Wilson TA. Action of the diaphragm on the rib cage. J Appl Physiol (1985) 2016;121:391-400. [PubMed]
- Mead J. Functional significance of the area of apposition of diaphragm to rib cage. Am Rev Respir Dis 1979;119:31-2. [PubMed]
- De Troyer A, Estenne M. Functional anatomy of the respiratory muscles. Clin Chest Med 1988;9:175-93. [PubMed]
- Hess DR. Respiratory Mechanics in Mechanically Ventilated Patients. Respir Care 2014;59:1773-94. [Crossref] [PubMed]
- Laporta D, Grassino A. Assessment of transdiaphragmatic pressure in humans. J Appl Physiol 1985;58:1469-76. [Crossref] [PubMed]
- Yoshida T, Amato MBP, Grieco DL, et al. Esophageal Manometry and Regional Transpulmonary Pressure in Lung Injury. Am J Respir Crit Care Med 2018;197:1018-26. [Crossref] [PubMed]
- Yoshida T, Brochard L. Ten tips to facilitate understanding and clinical use of esophageal pressure manometry. Intensive Care Med 2018;44:220-2. [Crossref] [PubMed]
- Mauri T, Yoshida T, Bellani G, et al. Esophageal and transpulmonary pressure in the clinical setting:meaning, usefulness and perspectives. Intensive Care Med 2016;42:1360-73. [Crossref] [PubMed]
- Akoumianaki E, Maggiore SM, Valenza F, et al. The application of esophageal pressure measurement in patients with respiratory failure. Am J Respir Crit Care Med 2014;189:520-31. [Crossref] [PubMed]
- De Troyer A, Boriek AM. Mechanics of the respiratory muscles. Compr Physiol 2011;1:1273-1300. [PubMed]
- Tobin MJ. Principles and Practise of Intensive Care Monitoring. 1st edition. The McGraw-Hill Companies, Inc., 1997.
- Grinnan DC, Truwit JD. Clinical review: Respiratory mechanics in spontaneous and assisted ventilation. Crit Care 2005;9:472-84. [Crossref] [PubMed]
- De Troyer A, Estenne M, Ninane V, et al. Transversus abdominis muscle function in humans. J Appl Physiol 1990;68:1010-6. [Crossref] [PubMed]
- Ninane V, Rypens F, Yernault JC, et al. Abdominal muscle use during breathing in patients with chronic airflow obstruction. Am Rev Respir Dis 1992;146:16-21. [Crossref] [PubMed]
- Dodd DS, Brancatisano T, Engel LA. Chest wall mechanics during exercise in patients with severe chronic air-flow obstruction. Am Rev Respir Dis 1984;129:33-8. [PubMed]
- Smith J, Bellemare F. Effect of lung volume on in vivo contraction characteristics of human diaphragm. J Appl Physiol 1987;62:1893-900. [Crossref] [PubMed]
- Aliverti A, Cala SJ, Duranti R, et al. Human respiratory muscle actions and control during exercise. J Appl Physiol 1997;83:1256-69. [Crossref] [PubMed]
- Cohen CA, Zagelbaum G, Gross D, et al. Clinical manifestations of inspiratory muscle fatigue. Am J Med 1982;73:308-16. [Crossref] [PubMed]
- Yang KL, Tobin MJ. A Prospective Study of Indexes Predicting the Outcome of Trials of Weaning from Mechanical Ventilation. N Engl J Med 1991;324:1445-50. [Crossref] [PubMed]
- Banner MJ, Kirby RR, Kirton OC, et al. Breathing frequency and pattern are poor predictors of work of breathing in patients receiving pressure support ventilation. Chest 1995;108:1338-44. [Crossref] [PubMed]
- Jubran A, Tobin MJ. Pathophysiologic basis of acute respiratory distress in patients who fail a trial of weaning from mechanical ventilation. Am J Respir Crit Care Med 1997;155:906-15. [Crossref] [PubMed]
- Sassoon CS, Light RW, Lodia R, et al. Pressure-time product during continuous positive airway pressure, pressure support ventilation, and T-piece during weaning from mechanical ventilation. Am Rev Respir Dis 1991;143:469-75. [Crossref] [PubMed]
- Mancebo J, Isabey D, Lorino H, et al. Comparative effects of pressure support ventilation and intermittent positive pressure breathing (IPPB) in non-intubated healthy subjects. Eur Respir J 1995;8:1901-9. [Crossref] [PubMed]
- Ballantine T V, Proctor HJ, Broussard ND, et al. The work of breathing: potential for clinical application and the results of studies performed on 100 normal males. Ann Surg 1970;171:590-4. [Crossref] [PubMed]
- Tobin MJ, Laghi F, Jubran A. Narrative review: ventilator-induced respiratory muscle weakness. Ann Intern Med 2010;153:240-5. [Crossref] [PubMed]
- Brochard L, Martin GS, Blanch L, et al. Clinical review: Respiratory monitoring in the ICU - a consensus of 16. Crit Care 2012;16:219. [Crossref] [PubMed]
- Banner MJ. Respiratory muscle loading and the work of breathing. J Cardiothorac Vasc Anesth 1995;9:192-204. [Crossref] [PubMed]
- Field S, Sanci S, Grassino A. Respiratory muscle oxygen consumption estimated by the diaphragm pressure-time index. J Appl Physiol 1984;57:44-51. [Crossref] [PubMed]
- Collett PW, Perry C, Engel LA. Pressure-time product, flow, and oxygen cost of resistive breathing in humans. J Appl Physiol 1985;58:1263-72. [Crossref] [PubMed]
- Nava S, Bruschi C, Fracchia C, et al. Patient-ventilator interaction and inspiratory effort during pressure support ventilation in patients with different pathologies. Eur Respir J 1997;10:177-83. [Crossref] [PubMed]
- Jubran A, Van de Graaff WB, Tobin MJ. Variability of patient-ventilator interaction with pressure support ventilation in patients with chronic obstructive pulmonary disease. Am J Respir Crit Care Med 1995;152:129-36. [Crossref] [PubMed]
- Dodd DS, Kelly S, Collett PW, et al. Pressure-time product, work rate, and endurance during resistive breathing in humans. J Appl Physiol 1988;64:1397-404. [Crossref] [PubMed]
- Dodd DS, Yarom J, Loring SH, et al. O2 cost of inspiratory and expiratory resistive breathing in humans. J Appl Physiol 1988;65:2518-23. [Crossref] [PubMed]
- Bellemare F, Grassino A. Effect of pressure and timing of contraction on human diaphragm fatigue. J Appl Physiol 1982;53:1190-5. [Crossref] [PubMed]
- McCool FD, McCann DR, Leith DE, et al. Pressure-flow effects on endurance of inspiratory muscles. J Appl Physiol 1986;60:299-303. [Crossref] [PubMed]
- Clanton TL, Ameredes BT, Thomson DB, et al. Sustainable inspiratory pressures over varying flows, volumes, and duty cycles. J Appl Physiol 1990;69:1875-82. [Crossref] [PubMed]
- Cabello B, Mancebo J. Work of breathing. Intensive Care Med 2006;32:1311-4. [Crossref] [PubMed]
- Cressoni M, Gotti M, Chiurazzi C, et al. Mechanical Power and Development of Ventilator-induced Lung Injury. Anesthesiology 2016;124:1100-8. [Crossref] [PubMed]
- Gattinoni L, Tonetti T, Cressoni M, et al. Ventilator-related causes of lung injury: the mechanical power. Intensive Care Med 2016;42:1567-75. [Crossref] [PubMed]
- Oppersma E, Hatam N, Doorduin J, et al. Functional assessment of the diaphragm by speckle tracking ultrasound during inspiratory loading. J Appl Physiol 2017;123:1063-70. [Crossref] [PubMed]
- Carrillo-Esper R, Perez-Calatayud AA, Arch-Tirado E, et al. Standardization of Sonographic Diaphragm Thickness Evaluations in Healthy Volunteers. Respir Care 2016;61:920-4. [Crossref] [PubMed]
- Santana PV, Prina E, Albuquerque ALP, et al. Identifying decreased diaphragmatic mobility and diaphragm thickening in interstitial lung disease: the utility of ultrasound imaging. J Bras Pneumol 2016;42:88-94. [Crossref] [PubMed]
- Thimmaiah VT. MJ G, Jain KP. Evaluation of Thickness of Normal Diaphragm by B Mode Ultrasound. Int J Contemp Med Res 2016;3:2658-60.
- Boussuges A, Gole Y, Blanc P. Diaphragmatic motion studied by M-mode ultrasonography. Chest 2009;135:391-400. [Crossref] [PubMed]
- De Troyer A, Farkas GA. Linkage between parasternals and external intercostals during resting breathing. J Appl Physiol 1990;69:509-16. [Crossref] [PubMed]
- De Troyer A, Peche R, Yernault JC, et al. Neck muscle activity in patients with severe chronic obstructive pulmonary disease. Am J Respir Crit Care Med 1994;150:41-7. [Crossref] [PubMed]
- Sinderby C, Navalesi P, Beck J, et al. Neural control of mechanical ventilation in respiratory failure. Nat Med 1999;5:1433-6. [Crossref] [PubMed]
- Carteaux G, Cordoba-Izquierdo A, Lyazidi A, et al. Comparison between Neurally Adjusted Ventilatory Assist and Pressure Support Ventilation Levels in Terms of Respiratory Effort. Crit Care Med 2016;44:503-11. [Crossref] [PubMed]
- Doorduin J, Sinderby CA, Beck J, et al. Automated patient-ventilator interaction analysis during neurally adjusted non-invasive ventilation and pressure support ventilation in chronic obstructive pulmonary disease. Crit Care 2014;18:550. [Crossref] [PubMed]
- Heunks LMA, Doorduin J, Van Der Hoeven JG. Monitoring and preventing diaphragm injury. Curr. Opin. Crit Care 2015;21:34-41. [Crossref] [PubMed]
- Kim MJ, Druz WS, Danon J, et al. Effects of lung volume and electrode position on the esophageal diaphragmatic EMG. J Appl Physiol Respir Environ Exerc Physiol 1978;45:392-8. [PubMed]
- Beck J, Sinderby C, Lindstrom L, et al. Effects of lung volume on diaphragm EMG signal strength during voluntary contractions. J Appl Physiol 1998;85:1123-34. [Crossref] [PubMed]
- Sinderby C, Spahija J, Beck J, et al. Diaphragm activation during exercise in chronic obstructive pulmonary disease. Am J Respir Crit Care Med 2001;163:1637-41. [Crossref] [PubMed]
- Sinderby C, Beck J, Spahija J, et al. Voluntary activation of the human diaphragm in health and disease. J Appl Physiol 1998;85:2146-58. [Crossref] [PubMed]
- Beck J, Sinderby C, Lindström L, et al. Diaphragm interference pattern EMG and compound muscle action potentials:effects of chest wall configuration. J Appl Physiol 1997;82:520-30. [Crossref] [PubMed]
- Liu L, Liu S, Xie J, et al. Assessment of patient-ventilator breath contribution during neurally adjusted ventilatory assist in patients with acute respiratory failure. Crit Care 2015;19:43. [Crossref] [PubMed]
- Grasselli G, Beck J, Mirabella L, et al. Assessment of patient-ventilator breath contribution during neurally adjusted ventilatory assist. Intensive Care Med 2012;38:1224-32. [Crossref] [PubMed]
- Haaksma M, Tuinman PR, Heunks L. Ultrasound to assess diaphragmatic function in the critically ill—a critical perspective. Ann Transl Med 2017;5:114. [Crossref] [PubMed]
- Zambon M, Beccaria P, Matsuno J, et al. Mechanical ventilation and diaphragmatic atrophy in critically ill patients: An ultrasound study. Crit Care Med 2016;44:1347-52. [Crossref] [PubMed]
- Grosu HB, Lee YI, Lee J, et al. Diaphragm muscle thinning in patients who are mechanically ventilated. Chest 2012;142:1455-60. [Crossref] [PubMed]
- Vivier E, Mekontso Dessap A, Dimassi S, et al. Diaphragm ultrasonography to estimate the work of breathing during non-invasive ventilation. Intensive Care Med 2012;38:796-803. [Crossref] [PubMed]
- Umbrello M, Formenti P, Longhi D, et al. Diaphragm ultrasound as indicator of respiratory effort in critically ill patients undergoing assisted mechanical ventilation: A pilot clinical study. Crit Care 2015;19:161. [Crossref] [PubMed]
- Goligher EC, Laghi F, Detsky ME, et al. Measuring diaphragm thickness with ultrasound in mechanically ventilated patients: feasibility, reproducibility and validity. Intensive Care Med 2015;41:642-9. [Crossref] [PubMed]
- Heunks L, Ottenheijm C. Diaphragm-Protective Mechanical Ventilation to Improve Outcomes in ICU Patients? Am J Respir Crit Care Med 2018;197:150-2. [Crossref] [PubMed]
- Powers SK, Wiggs MP, Sollanek KJ, et al. Ventilator-induced diaphragm dysfunction: cause and effect. Am J Physiol Regul Integr Comp Physiol 2013;305:R464-77. [Crossref] [PubMed]
- Vassilakopoulos T, Petrof BJ. Critical Care Perspective Ventilator-induced Diaphragmatic Dysfunction. Am J Respir Crit Care Med 2004;169:336-41. [Crossref] [PubMed]
- Hooijman PE, Beishuizen A, de Waard MC, et al. Diaphragm Fiber Strength Is Reduced in Critically Ill Patients and Restored by a Troponin Activator. Am J Respir Crit Care Med 2014;189:863-5. [Crossref] [PubMed]
- Hooijman PE, Beishuizen A, Witt CC, et al. Diaphragm muscle fiber weakness and ubiquitin-proteasome activation in critically ill patients. Am J Respir Crit Care Med 2015;191:1126-38. [Crossref] [PubMed]
- Demoule A, Molinari N, Jung B, et al. Patterns of diaphragm function in critically ill patients receiving prolonged mechanical ventilation: A prospective longitudinal study. Ann Intensive Care 2016;6:75. [Crossref] [PubMed]
- Jaber S, Petrof BJ, Jung B, et al. Rapidly Progressive Diaphragmatic Weakness and Injury during Mechanical Ventilation in Humans. Am J Respir Crit Care Med 2011;183:364-71. [Crossref] [PubMed]
- Goligher EC, Dres M, Fan E, et al. Mechanical Ventilation-induced Diaphragm Atrophy Strongly Impacts Clinical Outcomes. Am J Respir Crit Care Med 2018;197:204-13. [Crossref] [PubMed]
- van Hees HW, Schellekens WJM, Linkels M, et al. Plasma from septic shock patients induces loss of muscle protein. Crit Care 2011;15:R233. [Crossref] [PubMed]
- Annane D. What Is the Evidence for Harm of Neuromuscular Blockade and Corticosteroid Use in the Intensive Care Unit? Semin Respir Crit Care Med 2016;37:51-6. [Crossref] [PubMed]
- Puthucheary ZA, Rawal J, McPhail M, et al. Acute skeletal muscle wasting in critical illness. JAMA 2013;310:1591-600. [Crossref] [PubMed]
- Dres M, Goligher EC, Heunks LMA, et al. Critical illness-associated diaphragm weakness. Intensive Care Med 2017;43:1441-52. [Crossref] [PubMed]
- Bourenne J, Hraiech S, Roch A, et al. Sedation and neuromuscular blocking agents in acute respiratory distress syndrome. Ann Transl Med 2017;5:291. [Crossref] [PubMed]
- Gainnier M, Roch A, Forel JM, et al. Effect of neuromuscular blocking agents on gas exchange in patients presenting with acute respiratory distress syndrome*. Crit Care Med 2004;32:113-9. [Crossref] [PubMed]
- Forel JM, Roch A, Marin V, et al. Neuromuscular blocking agents decrease inflammatory response in patients presenting with acute respiratory distress syndrome*. Crit Care Med 2006;34:2749-57. [Crossref] [PubMed]
- Akoumianaki E, Lyazidi A, Rey N, et al. Mechanical ventilation-induced reverse-triggered breaths: A frequently unrecognized form of neuromechanical coupling. Chest 2013;143:927-38. [Crossref] [PubMed]
- Gerovasili V, Stefanidis K, Vitzilaios K, et al. Electrical muscle stimulation preserves the muscle mass of critically ill patients: A randomized study. Crit Care 2009;13:R161. [Crossref] [PubMed]
- Hirose T, Shiozaki T, Shimizu K, et al. The effect of electrical muscle stimulation on the prevention of disuse muscle atrophy in patients with consciousness disturbance in the intensive care unit. J Crit Care 2013;28:536.e1-7. [Crossref] [PubMed]
- Ayas NT, McCool FD, Gore R, et al. Prevention of human diaphragm atrophy with short periods of electrical stimulation. Am J Respir Crit Care Med 1999;159:2018-20. [Crossref] [PubMed]
- Doorduin J, Sinderby CA, Beck J, et al. Assisted Ventilation in Patients with Acute Respiratory Distress Syndrome. Anesthesiology 2015;123:181-90. [Crossref] [PubMed]
- Slutsky AS, Ranieri VM. Ventilator-Induced Lung Injury. N Engl J Med 2013;369:2126-36. [Crossref] [PubMed]
- Yoshida T, Roldan R, Beraldo MA, et al. Spontaneous Effort During Mechanical Ventilation. Crit Care Med 2016;44:e678-88. [Crossref] [PubMed]
- Doorduin J, Nollet JL, Roesthuis LH, et al. Partial Neuromuscular Blockade during Partial Ventilatory Support in Sedated Patients with High Tidal Volumes. Am J Respir Crit Care Med 2017;195:1033-42. [Crossref] [PubMed]
- Gattinoni L, Carlesso E, Caironi P. Stress and strain within the lung. Curr Opin Crit Care 2012;18:42-7. [Crossref] [PubMed]
- Mellott KG, Grap MJ, Munro CL, et al. Patient ventilator asynchrony in critically ill adults: Frequency and types. Heart Lung 2014;43:231-43. [Crossref] [PubMed]
- Talmor D, Sarge T, Malhotra A, et al. Mechanical ventilation guided by esophageal pressure in acute lung injury. N Engl J Med 2008;359:2095-104. [Crossref] [PubMed]
- Gea J, Zhu E, Gáldiz JB, et al. Functional consequences of eccentric contractions of the diaphragm. Arch Bronconeumol 2009;45:68-74. [PubMed]
- Watchko JF, Johnson BD, Gosselin LE, et al. Age-related differences in diaphragm muscle injury after lengthening activations. J Appl Physiol 1994;77:2125-33. [Crossref] [PubMed]
- Zhu E, Petrof BJ, Gea J, et al. Diaphragm muscle fiber injury after inspiratory resistive breathing. Am J Respir Crit Care Med 1997;155:1110-6. [Crossref] [PubMed]
- Jiang TX, Reid WD, Road JD. Delayed diaphragm injury and diaphragm force production. Am J Respir Crit Care Med 1998;157:736-42. [Crossref] [PubMed]
- Ebihara S, Hussain SNA, Danialou G, et al. Mechanical ventilation protects against diaphragm injury in sepsis: Interaction of oxidative and mechanical stresses. Am J Respir Crit Care Med 2002;165:221-8. [Crossref] [PubMed]
- Orozco-Levi M, Lloreta J, Minguella J, et al. Injury of the human diaphragm associated with exertion and chronic obstructive pulmonary disease. Am J Respir Crit Care Med 2001;164:1734-9. [Crossref] [PubMed]
- Doorduin J, Van Hees HWH, Van Der Hoeven JG, et al. Monitoring of the respiratory muscles in the critically Ill. Am J Respir Crit Care Med 2013;187:20-7. [Crossref] [PubMed]