Effect of acellular nerve scaffold containing human umbilical cord-derived mesenchymal stem cells on nerve repair and regeneration in rats with sciatic nerve defect
Introduction
Only some organs have intrinsic repair and regenerative capacity in the human body, and different organs often show different rates of repair and regeneration, such as the peripheral nervous system (1). Sciatic nerve injury is a common peripheral neuropathy usually caused by trauma, inducing the loss of motor and sensory functions (2,3). Patients suffering from such injury could experience symptoms, such as pain, muscle weakness, motor disturbance, and even long-term disability (2,3). Unfortunately, the ability of the sciatic nerve to generate and repair is limited, and its functional recovery is poor (4). The slow rate of sciatic nerve regeneration could result in permanent damage to the structure and function of its associated organs, and it can be a permanent complication before reinnervation of regenerating axons (5). In addition, peripheral nerve defects could lead to a gap between the nerve ends, and destruction of myelin sheath and axons. One study has tried to accelerate nerve regeneration in a variety of ways, including drug therapy, surgical techniques, physical therapy, rehabilitation techniques, and stem cell and biomaterial applications (6). At present, autologous transplantation is often used to repair peripheral nerves and sciatic nerves clinically, but it is difficult to preserve the transplanted nerve due to its low survival rate at the recipient site (7). Allotransplantation has also been tested in clinical trials, and blood vessels, degenerated muscle fibers, and venous catheters can be used as materials for nerve repair; however, the success rate is very low and the results are poor (8). The failure of these methods can be attributed to multiple factors, such as the inability of neurotrophic factors produced by the proximal never stump to reach the distal stump, the lack of supporting structures, and the absence of Schwann cells (SCs) (9). Therefore, at present, most researchers regard tissue engineering of peripheral nerves as a perspective strategy to create a better environment for reconstructing damaged nerves.
Current research on peripheral nerve tissue engineering has focused on the implantation of ideal cultured “seed cells” into appropriate scaffolds. Acellular nerve allografts have been used successfully and with increasing frequency to reconstruct nerve injuries (10). In the process of nerve defect repair, seed cells can promote the regeneration of myelinated nerve fibers and axons, and can secrete a large number of cell growth factors to improve the microenvironment of nerve repair (11). Allogeneic acellular nerve scaffold (ANS) has incomparable advantages over other nerve substitutes, and can serve as an effective regenerative material that can preserve the 3D spatial structure of natural nerves (12). The commonly used seed cells include bone marrow-derived mesenchymal stem cells (MSCs), adipose-derived MSCs, neural stem cells, and SCs (13). Zhou et al. found that the transplantation of ANS filled with bone marrow stromal cells and SCs promoted the recovery of sciatic nerve function in rats (14). An et al. found that adipose stem cells proliferate faster in vitro, while bone marrow MSCs have higher transformation potential to chondrocytes (15). Qiao et al. found that a myroilysin-treated acellular nerve graft with homologous dental pulp stem cells enhanced sciatic nerve regeneration in New Zealand white rabbits (16). In their study, Zheng et al. demonstrated that allogeneic acellular nerve graft with platelet-rich plasma improved nerve regeneration after surgical repair and prevented muscle atrophy (17). Composite chitosan scaffolds loaded with controlled release of nerve growth factor can promote neuronal survival and axonal regeneration after short-segment sciatic nerve defects (18). Collectively, filling ANS with stem cells or a mixture rich in growth factors can significantly enhance repair ability after sciatic nerve injury. According to one existing report, MSCs have the ability to self-renew and the potential to differentiate into various lineages, so they have been widely applied in the treatment of chronic diseases, osteoarthritis, and cartilage repair (19). Compared with MSCs from other sources, human umbilical cord-derived MSCs (hUC-MSCs) have fewer social ethical problems and low immunogenicity, so hUC-MSCs are a suitable choice for allogeneic transplantation (20). It has also been pointed out that a longitudinal-oriented collagen conduit loaded with hUC-MSCs to bridge the sciatic nerve can promote sciatic nerve regeneration and accelerate functional recovery (21). However, there are no reports studying the effect of hUC-MSCs in ANS filled with hUC-MSCs on repairing the sciatic nerve. Therefore, in the present study, we constructed a rat model of sciatic nerve defects and ANS filled with hUC-MSCs, and further explored the repairing effect of ANS after sciatic nerve injury. The aim of the present study was provide new methods and effective experimental data for the clinical treatment of sciatic nerve defects. We present the following article in accordance with the ARRIVE reporting checklist (available at https://atm.amegroups.com/article/view/10.21037/atm-22-1578/rc).
Methods
Experimental animals
Thirty healthy adult female Sprague-Dawley (SD) rats weighing 200–250 g were purchased from Beijing Vital River Laboratory Animal Technology (Beijing, China). They were housed in an environment with a temperature of 22 ℃ and 50% humidity for 7 days, and then used for subsequent experiments. A protocol was prepared before the study without registration. Animal experiments were granted by committee board of the Children’s Hospital of Fudan University and National Children’s Medical Center, in compliance with the Children’s Hospital of Fudan University and National Children’s Medical Center’s guideline for the care and use of animals.
ANS preparation
A 5-cm sciatic nerve trunk was removed from 6 rats as a raw material. Subsequently, fat and connective tissue attached to the sciatic nerve were removed. ANS was prepared using the lyophilization + enzymatic method, and to select the optimal treatment time, the removed sciatic nerve trunks were randomly divided into five groups (A, B, C, D, and E). Group A was treated with the following steps: (I) step 1: 10 mM of hypotonic tris buffer + serine protease inhibitor [0.05% phenylmethylsulfonyl fluoride (PMSF) solution: 35 mL/L 50 mL ethanol + 25 mg PMSF], 12 h, ambient temperature; (II) step 2: phosphate-buffered saline (PBS) solution + 0.05% PMSF solution + 0.05% trypsin, 5 h, ambient temperature; (III) step 3: PBS solution + 90 U/mL DNase, 5 h, 37 ℃; and (IV) step 4: rinsed with PBS for 48 h and stored in sterile PBS + 5 mL/L solution containing penicillin (10,000 U/mL) and streptomycin solution (10,000 mg/mL) at 4 ℃. Compared with group A, the incubation time was 24 h for step 1 in group B, 10 h for step 3 in group C, 24 h for step 1, and 10 h for step 3 in group D. The remaining steps of groups B, C, and D were kept consistent with those of group A. In addition, group E was normal sciatic nerve without other treatment.
Isolation and culture of hUC-MSCs
hUC-MSCs were isolated from Wharton’s jelly of human umbilical cord that was collected from the Children’s Hospital of Fudan University and National Children’s Medical Center. The study was conducted in accordance with the Declaration of Helsinki (as revised in 2013). Informed consent was obtained from parturient volunteers, and all procedures were approved by the ethics committee of the Children’s Hospital of Fudan University and National Children’s Medical Center (No. 2022-59). Approximately 8 cm-long umbilical cords were isolated in a sterile setting and soaked in sterile PBS. After removal of blood vessels and other tissues, Wharton’s jelly was cut into 1–3-mm3 pieces and adhered to the bottom of the dish. Subsequently, a complete medium (MesenGro hMSC medium; StemRD, Guangzhou, China) was added into the dish, and half of the medium was changed every 3 days. After 7 days of culture, the debris was removed and the medium was changed. Approximately 14 days later, hUC-MSCs were observed to gradually grow into a dense population, and then when confluence reached 80–90%, the cells were passaged using 0.25% trypsin-ethylenediaminetetraacetic acid (trypsin-EDTA) (Sigma-Aldrich, Shanghai, China). Those after passage 3 were used for subsequent experiments.
Co-culture of hUC-MSCs and ANS
ANS (5-mm length) was prepared using the group A method of and placed in a culture dish and soaked overnight in complete Dulbecco’s modified Eagle’s medium/F-12 medium. Then 106/100 µL of hUC-MSCs was added into ANS and cultured with ANS for 1 week to obtain the ANS-hUC-MSCs complex.
Construction of a rat model of sciatic nerve defect
Construction of sciatic nerve defect model (22). Twenty-four rats were randomly divided into four groups. In the control group, the rats were anesthetized and the right sciatic nerve was exposed, and then the wound was closed. In the autograft group, the right sciatic nerve was exposed, and then the tibial and common peroneal nerves of the sciatic trunk were cut at the popliteal fossa, resulting in a nerve defect of about 1 cm in length. Finally, an inverted anastomosis of the proximal and distal sciatic nerve ends was carried out, followed by fixation using atraumatic sutures. In the ANS group, the proximal and distal sciatic nerve ends were anastomosed to ANS. In the ANS-hUC-MSC group, the proximal and distal sciatic nerve ends were anastomosed to the scaffold containing hUC-MSCs. After 8 weeks of feeding, the rats were killed to isolate the sciatic nerve and thigh muscle. The muscle tissue was weighed, and then the recovery rate of muscle wet weight was calculated (muscle wet weight recovery rate = wet weight of the muscle on the experimental side/wet weight of the muscle on the healthy side).
Hematoxylin-eosin (HE) staining
The prepared ANS was fixed using 4% paraformaldehyde, and then tissue samples were embedded and sectioned at a thickness of 4 µm. The sections were rinsed 3 times with PBS for 10 min each. The next steps included staining with hematoxylin at ambient temperature for 5 min, differentiation with hydrochloric acid alcohol, and bluing in 1% (v/v) ammonia water. The samples were then rinsed with tap water and stained with eosin for 30 s. Finally, the sections were mounted using neutral resin after dehydration with alcohol and clearing with xylene. Histopathological morphology was observed under a biomicroscope.
Luxol fast blue (LFB) staining
ANS and sciatic nerve tissues were removed and fixed in paraformaldehyde, followed by paraffin embedding and fixation of the site of lumbar enlargement of the spinal cord. The samples were then sliced at a thickness of about 4 µm, followed by deparaffinization with xylene. Staining was performed using LFB staining solution. Subsequently, the sections were rinsed, differentiated, dehydrated, and finally mounted using neutral resin. A light microscope was used to observe the degree of demyelination of the nerve, as well as the degree of remyelination in cross-sections of regenerated sciatic nerve at the proximal ends of the graft.
Hydroxyproline (HYP) testing
A portion of ANS from each group was placed in a homogenization tube, and then 1 mL of precooled PBS buffer was added. The tissues were disrupted in a cryogenic homogenizer. The obtained homogenate was centrifuged at 12,000 ×g for 10 min at 4 ℃, followed by removal of cell debris. Finally, the supernatant was collected and HYP levels were measured according to the instructions of the HYP assay kit (Beyotime, Shanghai, China).
Scanning electron microscopy
Sciatic nerve tissues were taken and fixed using 4% paraformaldehyde. The tissues were then fixed using 1% OsO4 and dehydrated by gradient alcohol and acetone. They were then polymerized at 60 ℃ for 72 h after resin infiltration. An electron microscope was used to photograph and observe the nerve fiber morphological structure of nerve fibers and the nerve fibers in the cross-section of the regenerated sciatic nerve at the distal end of the graft.
Mechanical testing
Mechanical testing (23) of the sciatic nerve was performed using an Instron 505 universal testing machine with an initial length of 10 mm. The specimen was pulled off at a loading rate of 5 mm/min, and the elastic modulus (MPa), maximum tensile load (N), maximum displacement (mm), and ultimate stress (MPa) were recorded.
Flow cytometry
Third-passage hUC-MSCs were collected, and the surface markers of stem cells were identified by flow cytometry. According to the operating procedures, hUC-MSC were incubated with primary antibodies CD29, CD44, and CD34, followed by secondary antibodies labeled with phycoerythrin. Finally, cell immunofluorescence was tested using a flow cytometer (Beckman Coulter, Suzhou, Chima).
Electrophysiological examination
Electrophysiological examination was performed on rats 8 weeks after surgery. Stimulation electrodes were placed 0.5 cm proximal to the sciatic nerve graft, and the recording electrodes placed in the gastrocnemius muscle 10 mm below the tibial tubercle to record compound muscle action potentials (CMAP) and to calculate nerve conduction velocity (NCV) of the regenerated sciatic nerve.
Masson staining
The rat muscle tissues were fixed using 4% paraformaldehyde and dehydrated through gradient alcohols. Cleaning in xylene and preparation of paraffin sections were then carried out. After deparaffinization, the nucleus was stained with the prepared Weigert’s iron hematoxylin solution for 7 min. On completion of staining, tissues were treated with the following steps: differentiation using acidic differentiation solution for 10 s, bluing for 4 min, and staining using ponceau-fuchsin staining solution for 8 min. The weak acid working solution was then used for 1 min rinsing, phosphomolybdic acid solution for 2 min wash, and then weak acid working solution for 1 min wash. Subsequently, the tissues were stained with aniline blue staining solution for 1 min, rapidly dehydrated using 95% (v/v) ethanol, and mounted using neutral resin. Finally, the cross-section of gastrocnemius muscle fibers on the experimental side was observed under a microscope.
Statistical analysis
All data were analyzed using SPSS 26.0 (IBM, Armonk, NY, USA), and comparisons between two groups were analyzed using the independent samples t-test. Results were expressed as mean ± standard deviation. P<0.05 indicated statistical significance.
Results
Selection of optimal time for ANS preparation
The optimal time for ANS preparation was selected by the results of HE staining, LFB staining, scanning electron microscopy, mechanical examination, and HYP testing. According to HE staining results, group A showed more and neatly arranged cells, while groups A, B, C, and D had nuclei reduced to varying degrees and disorganized cells (Figure 1A). The results of LFB staining and electron microscopy showed that group E had more myelin sheaths, thicker sheath walls, and significant axons, while the remaining four groups presented residues of axons, myelin sheaths, and other structures. Among the latter four groups, group A had the least number of myelin sheaths, loose and porous structure, and significantly thinner sheath walls (Figure 1B,1C). In addition, compared with group E, the contents of HYP in groups C and D were significantly decreased, but that in groups A and B had no significant changes (Figure 1D). Further mechanical testing results also indicated that group E had the lowest values of elastic modulus, maximum tensile load, maximum displacement, and ultimate stress; group A had lower values of elastic modulus, maximum tensile load, maximum displacement. and ultimate stress than groups B, C, and D (Figure 1E). These findings indicated that ANS prepared in group A had the best performance.
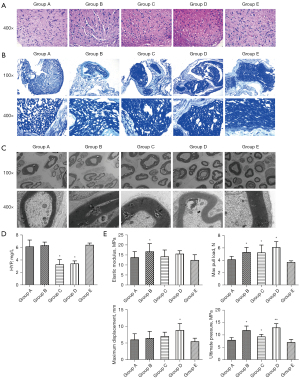
ANS containing hUC-MSCs significantly ameliorates sciatic nerve tissue injury
Isolated and cultured hUC-MSCs were identified, and positive results of hUC-MSC surface markers CD29 and CD44 and a negative result of CD34 were obtained (Figure 2A), indicating successful isolation of hUC-MSCs. The effect of ANS containing hUC-MSCs was further evaluated. According to LFB staining and scanning electron microscopy, the number of myelin sheaths was significantly decreased and the sheath wall was thinned in the ANS group compared with the control group, suggesting that the nerve tissue was damaged. After further treatment with ANS containing hUC-MSCs, we found marked increases of the proportion of myelin in the tissue and the myelin thickness, similar to the autograft group (Figure 2B,2C). Collectively, ANS containing hUC-MSCs could significantly ameliorate tissue injury in a rat model of sciatic nerve injury.
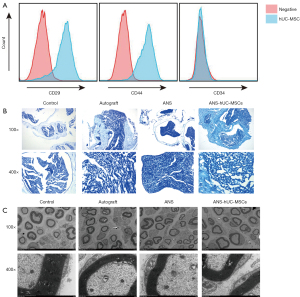
ANS containing hUC-MSCs effectively restores sciatic nerve function
The effect of ANS containing hUC-MSCs on sciatic nerve function in rats was further investigated. The results of electrophysiological examination showed that compared with the control group, the CMAP and NCV of the ANS-hUC-MSCs group, autograft group, and ANS group had a significant reduction. Compared with the ANS group, a marked increase of these two indicators were found in the ANS-hUC-MSCs group and autograft group (Figure 3A). In addition, the muscle wet weight in the autograft group and ANS-hUC-MSCs group was greater than that in the ANS group (Figure 3B). Further Masson staining results showed that the cells in the ANS group appeared to be loosely arranged, with a large number of collagen fibers and obvious atrophy. After treatment of autograft or ANS-hUC-MSCs, the cells had a neat arrangement, a reduction of collagen fibers, and a certain degree of tissue recovery (Figure 3C). Taken together, ANS containing hUC-MSCs had a greater effect on the recovery of sciatic nerve function.
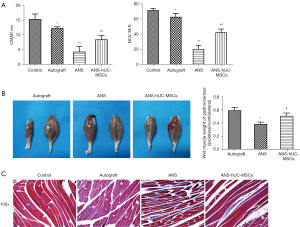
Discussion
Peripheral nerve defects are common in clinical practice, but nerve injury repair is difficult in surgery (24). Autologous nerve transplantation is considered to be the most effective method for nerve injury repair, but its success rate is still unsatisfactory (25). ANS is emerging as a promising alternative to repair nerve injury and has been widely used in the treatment of peripheral nerve defects (26). However, the location and length of nerve injury and the length of ANS still affect the effect of nerve repair (27). In addition, traditional ANS has a dense structure with low porosity and a small void diameter, so it cannot meet the requirements for cell seeding and its application is limited (28). Therefore, it is important to construct a novel ANS with a better structure. The key to the preparation of scaffolds is in the removal of cells carried by major histocompatibility complexes and the avoidance of immune rejection (29). During the preparation, axonal regeneration is affected by the degree of decellularization and demyelination, as well as the integrity of nerve fiber and laminin activity (30). If these influencing factors are destroyed, axonal regeneration will be delayed and destroy the nerve basement membrane scaffold (31). There have been one study to improve the preparation of ANS and construct novel scaffolds superior to traditional ones. Performance can be evaluated by indicators, including maximum tensile load (reflecting the strength of the nerve), ultimate stress (the maximum stress acting on the unit area), and elastic modulus (representing antideformation and fracture resistance of the nerve, an intrinsic parameter of the nerve) (23). HYP reflects vascular stromal cell composition (32). The acellular matrix requires the removal of muscle fibers and cellular components, and the retention of an intact extracellular matrix (33). In the present study, developed an improved ANS preparation method, that is, 10 mM hypotonic tris buffer + serine protease inhibitor for 12 h, 0.05% PMSF + 0.05% trypsin for 5 h, then 90 U/mL DNase for 5 h, and PBS rinsing for 48 h. After these procedures, the biomechanical parameters and HYP levels of ANS were found to be less different from those of the normal nerve, and its characterization was most suitable for cell implantation. It has been reported that the lyophilization + enzymatic method can preserve the mechanical properties of normal sciatic nerves, and therefore obtain ideal acellular and unmyelinated scaffold materials (34). These results are consistent with ours. Compared with other reported methods (35), our method is simpler and has the advantage of low cost.
According to clinical experience, once the peripheral nervous system is damaged, its integrity is difficult to restore, and its self-repair function is low (36). These factors lead to great difficulties in the treatment of nerve defects. Stem cell-based therapy has attracted wide attention in recent years due to vigorous viability and strong differentiation ability of stem cells (37). However, stem cells can be derived from a wide range of sources, such as bone marrow and adipose tissue, and stem cells from different sources have different proliferation and differentiation abilities. Compared with stem cells from other sources, it is easier to obtain hUC-MSCs because of the rich number of discarded umbilical cords, and to isolate and collect hUC-MSCs from the umbilical cord (38). In addition, hUC-MSCs have faster proliferation and are non-tumorigenic, because hUC-MSCs cells are more primitive than other MSCs and are considered to have higher differentiation potential (39). These results suggest that hUC-MSCs are ideal raw materials for cell engineering. In a related study by Wang et al., transplantation of hUC-MSCs with overexpression of brain-derived neurotrophic factor improved motor performance and prolonged survival in amyotrophic lateral sclerosis rats (40). Shi et al. found that in rats with traumatic brain injury, transplantation of hUC-MSCs with activated astrocytes could promote tissue regeneration in moderate-sized or large-lesion cavities and improve brain tissue injury (41). Sciatic nerve injury can lead to muscle paralysis and motor disturbance (42). The findings of the present study indicated that ANS containing hUC-MSCs could significantly promote the regeneration of myelin and axons, improve muscle atrophy, and restore sciatic nerve function. Such effects indicate that hUC-MSCs have ideal neural repair properties.
Conclusions
The present study provides a preparation method for ANS with better results than the traditional method. The novel method can effectively remove cells and myelin, and maintain the integrity and mechanical properties of nerve fiber. We also found that the combination of hUC-MSCs with ANS promotes repair and regeneration of the sciatic nerve. However, the findings of the present study failed to further reveal its mechanism. Further exploration on the mechanism is expected to comprehensively explain the function of ANS containing hUC-MSCs, and provide more data to support its clinical application.
Acknowledgments
Funding: This work was supported by The Clinical Center of Birth Defects (EK2022ZX03).
Footnote
Reporting Checklist: The authors have completed the ARRIVE reporting checklist. Available at https://atm.amegroups.com/article/view/10.21037/atm-22-1578/rc
Data Sharing Statement: Available at https://atm.amegroups.com/article/view/10.21037/atm-22-1578/dss
Conflicts of Interest: All authors have completed the ICMJE uniform disclosure form (available at https://atm.amegroups.com/article/view/10.21037/atm-22-1578/coif). The authors have no conflicts of interest to declare.
Ethical Statement: The authors are accountable for all aspects of the work in ensuring that questions related to the accuracy or integrity of any part of the work are appropriately investigated and resolved. Animal experiments were granted by committee board of the Children’s Hospital of Fudan University and National Children’s Medical Center, in compliance with the Children’s Hospital of Fudan University and National Children’s Medical Center guideline for the care and use of animals. The study was conducted in accordance with the Declaration of Helsinki (as revised in 2013). Informed consent was obtained from parturient volunteers, and all procedures were approved by the ethics committee of the Children’s Hospital of Fudan University and National Children’s Medical Center (No. 2022-59).
Open Access Statement: This is an Open Access article distributed in accordance with the Creative Commons Attribution-NonCommercial-NoDerivs 4.0 International License (CC BY-NC-ND 4.0), which permits the non-commercial replication and distribution of the article with the strict proviso that no changes or edits are made and the original work is properly cited (including links to both the formal publication through the relevant DOI and the license). See: https://creativecommons.org/licenses/by-nc-nd/4.0/.
References
- Jaźwińska A, Sallin P. Regeneration versus scarring in vertebrate appendages and heart. J Pathol 2016;238:233-46. [Crossref] [PubMed]
- Dadon-Nachum M, Melamed E, Offen D. Stem cells treatment for sciatic nerve injury. Expert Opin Biol Ther 2011;11:1591-7. [Crossref] [PubMed]
- Ciaramitaro P, Mondelli M, Logullo F, et al. Traumatic peripheral nerve injuries: epidemiological findings, neuropathic pain and quality of life in 158 patients. J Peripher Nerv Syst 2010;15:120-7. [Crossref] [PubMed]
- Silver J, Schwab ME, Popovich PG. Central nervous system regenerative failure: role of oligodendrocytes, astrocytes, and microglia. Cold Spring Harb Perspect Biol 2014;7:a020602. [Crossref] [PubMed]
- Cattin AL, Lloyd AC. The multicellular complexity of peripheral nerve regeneration. Curr Opin Neurobiol 2016;39:38-46. [Crossref] [PubMed]
- Gordon T. Electrical Stimulation to Enhance Axon Regeneration After Peripheral Nerve Injuries in Animal Models and Humans. Neurotherapeutics 2016;13:295-310. [Crossref] [PubMed]
- Snyder AK, Fox IK, Nichols CM, et al. Neuroregenerative effects of preinjury FK-506 administration. Plast Reconstr Surg 2006;118:360-7. [Crossref] [PubMed]
- Martínez de Albornoz P, Delgado PJ, Forriol F, et al. Non-surgical therapies for peripheral nerve injury. Br Med Bull 2011;100:73-100. [Crossref] [PubMed]
- Lundborg G, Kanje M. Bioartificial nerve grafts. A prototype. Scand J Plast Reconstr Surg Hand Surg 1996;30:105-10. [Crossref] [PubMed]
- Peters BR, Wood MD, Hunter DA, et al. Acellular Nerve Allografts in Major Peripheral Nerve Repairs: An Analysis of Cases Presenting With Limited Recovery. Hand (N Y) 2021; Epub ahead of print. [Crossref] [PubMed]
- Santosa KB, Jesuraj NJ, Viader A, et al. Nerve allografts supplemented with schwann cells overexpressing glial-cell-line-derived neurotrophic factor. Muscle Nerve 2013;47:213-23. [Crossref] [PubMed]
- Tang P, Chauhan A. Decellular Nerve Allografts. J Am Acad Orthop Surg 2015;23:641-7. [Crossref] [PubMed]
- Fan L, Yu Z, Li J, et al. Schwann-like cells seeded in acellular nerve grafts improve nerve regeneration. BMC Musculoskelet Disord 2014;15:165. [Crossref] [PubMed]
- Zhou LN, Zhang JW, Liu XL, et al. Co-Graft of Bone Marrow Stromal Cells and Schwann Cells Into Acellular Nerve Scaffold for Sciatic Nerve Regeneration in Rats. J Oral Maxillofac Surg 2015;73:1651-60. [Crossref] [PubMed]
- An RZ, Zhao JY, Wang ZJ. Chondrogenic potential of adipose-derived stem cells versus bone marrow mesenchymal stem cells. Journal of Clinical Rehabilitative Tissue Engineering Research 2013;17:5793-8.
- Qiao W, Lu L, Wu G, et al. DPSCs seeded in acellular nerve grafts processed by Myroilysin improve nerve regeneration. J Biomater Appl 2019;33:819-33. [Crossref] [PubMed]
- Zheng C, Zhu Q, Liu X, et al. Improved peripheral nerve regeneration using acellular nerve allografts loaded with platelet-rich plasma. Tissue Eng Part A 2014;20:3228-40. [Crossref] [PubMed]
- Liu FD, Duan HM, Hao F, et al. Biomimetic chitosan scaffolds with long-term controlled release of nerve growth factor repairs 20-mm-long sciatic nerve defects in rats. Neural Regen Res 2022;17:1146-55. [Crossref] [PubMed]
- Najar M, Raicevic G, Fayyad-Kazan H, et al. Mesenchymal stromal cells and immunomodulation: A gathering of regulatory immune cells. Cytotherapy 2016;18:160-71. [Crossref] [PubMed]
- Bongso A, Fong CY. The therapeutic potential, challenges and future clinical directions of stem cells from the Wharton's jelly of the human umbilical cord. Stem Cell Rev Rep 2013;9:226-40. [Crossref] [PubMed]
- Cui Y, Yao Y, Zhao Y, et al. Functional collagen conduits combined with human mesenchymal stem cells promote regeneration after sciatic nerve transection in dogs. J Tissue Eng Regen Med 2018;12:1285-96. [Crossref] [PubMed]
- Zhou LN, Cui XJ, Su KX, et al. Repairment of sciatic nerve defect by Schwann cell of adult rat combined with acellular nerve grafts. Organ Transplantation 2015;6:268-72.
- Yang Z, Ma XL, Li XL, et al. Effects of Different Acellular Methods on Biomechanical Properties of Peripheral Nerve. Chinese Journal of Biomedical Engineering 2011;30:155-9.
- Lee J, Choi J, Kang S, et al. Hepatogenic Potential and Liver Regeneration Effect of Human Liver-derived Mesenchymal-Like Stem Cells. Cells 2020;9:1521. [Crossref] [PubMed]
- Scholz T, Krichevsky A, Sumarto A, et al. Peripheral nerve injuries: an international survey of current treatments and future perspectives. J Reconstr Microsurg 2009;25:339-44. [Crossref] [PubMed]
- Weber RV, Mackinnon SE. Bridging the neural gap. Clin Plast Surg 2005;32:605-16. viii. [Crossref] [PubMed]
- Xiang FF, Yang YK. Research progress of acellular nerve scaffold in the repair of peripheral nerve defects. Sichuan Medical Journal 2016;37:1403-6.
- Marconi S, Castiglione G, Turano E, et al. Human adipose-derived mesenchymal stem cells systemically injected promote peripheral nerve regeneration in the mouse model of sciatic crush. Tissue Eng Part A 2012;18:1264-72. [Crossref] [PubMed]
- Gilbert TW, Sellaro TL, Badylak SF. Decellularization of tissues and organs. Biomaterials 2006;27:3675-83. [PubMed]
- Nagao RJ, Lundy S, Khaing ZZ, et al. Functional characterization of optimized acellular peripheral nerve graft in a rat sciatic nerve injury model. Neurol Res 2011;33:600-8. [Crossref] [PubMed]
- Sun M, Wang X, Zhao B. Quality estimation and influence factors of the larger chemically acellular nerve allografts in vitro. Zhongguo Xiu Fu Chong Jian Wai Ke Za Zhi 2006;20:779-82. [PubMed]
- Hanai H, Jacob G, Nakagawa S, et al. Potential of Soluble Decellularized Extracellular Matrix for Musculoskeletal Tissue Engineering - Comparison of Various Mesenchymal Tissues. Front Cell Dev Biol 2020;8:581972. [Crossref] [PubMed]
- Qing Q, Qin T. Optimal method for rat skeletal muscle decellularization. Zhongguo Xiu Fu Chong Jian Wai Ke Za Zhi 2009;23:836-9. [PubMed]
- Wang Q, Zhang C, Zhang L, et al. The preparation and comparison of decellularized nerve scaffold of tissue engineering. J Biomed Mater Res A 2014;102:4301-8. [Crossref] [PubMed]
- Zhao B, Sun X, Li X, et al. Improved preparation of acellular nerve scaffold and application of PKH26 fluorescent labeling combined with in vivo fluorescent imaging system in nerve tissue engineering. Neurosci Lett 2013;556:52-7. [Crossref] [PubMed]
- Grinsell D, Keating CP. Peripheral nerve reconstruction after injury: a review of clinical and experimental therapies. Biomed Res Int 2014;2014:698256. [Crossref] [PubMed]
- Shen W, Chen J, Zhu T, et al. Osteoarthritis prevention through meniscal regeneration induced by intra-articular injection of meniscus stem cells. Stem Cells Dev 2013;22:2071-82. [Crossref] [PubMed]
- Chen MY, Lie PC, Li ZL, et al. Endothelial differentiation of Wharton's jelly-derived mesenchymal stem cells in comparison with bone marrow-derived mesenchymal stem cells. Exp Hematol 2009;37:629-40. [Crossref] [PubMed]
- Fong CY, Chak LL, Biswas A, et al. Human Wharton's jelly stem cells have unique transcriptome profiles compared to human embryonic stem cells and other mesenchymal stem cells. Stem Cell Rev Rep 2011;7:1-16. [Crossref] [PubMed]
- Wang J, Hu W, Feng Z, et al. BDNF-overexpressing human umbilical cord mesenchymal stem cell-derived motor neurons improve motor function and prolong survival in amyotrophic lateral sclerosis mice. Neurol Res 2021;43:199-209. [Crossref] [PubMed]
- Shi W, Huang CJ, Xu XD, et al. Transplantation of RADA16-BDNF peptide scaffold with human umbilical cord mesenchymal stem cells forced with CXCR4 and activated astrocytes for repair of traumatic brain injury. Acta Biomater 2016;45:247-61. [Crossref] [PubMed]
- Ganguly A, McEwen C, Troy EL, et al. Recovery of sensorimotor function following sciatic nerve injury across multiple rat strains. J Neurosci Methods 2017;275:25-32. [Crossref] [PubMed]
(English Language Editor: R. Scott)