The critical role and molecular mechanisms of ferroptosis in antioxidant systems: a narrative review
Introduction
Cell death is the irreversible cessation of cellular activity and can be categorized into various types based on morphological, biochemical, and regulatory features, including apoptosis, necrosis, and autophagy. Ferroptosis was first discovered as a new form of regulatory cell death in 2012 and differs from other cell death pathways in terms of morphology, biochemistry, and regulatory mechanisms (1). It is characterized by the loss of plasma membrane integrity, cytoplasmic swelling, mitochondrial atrophy, rupture of the mitochondrial outer membrane, and moderate chromatin condensation (2). Its biochemical characteristics are cystine deficiency, glutathione (GSH) depletion, and glutathione peroxidase 4 (GPX4) inactivation, though its underlying mechanisms have not yet been fully elucidated (3). Research on the mechanisms of ferroptosis mainly focus on lipid peroxidation and iron loading. Great progress has been achieved in the System Xc-GSH-GPX4 pathway, which is one of the most important antioxidant systems protecting cells from ferroptosis. With the deepening of research, other antioxidant systems have also been implicated in regulating ferroptosis, including the transsulfuration pathway, mevalonate (MVA) pathway, ferroptosis inhibitory protein 1 (FSP1)-Coenzyme Q10 (CoQ10) pathway, dihydroorotate dehydrogenase (DHODH)-dihydroubiquione (CoQH2) pathway, and GTP cyclohydrolase-1 (GCH1)-tetrahydrobiopterin (BH4) pathway (Figure 1).
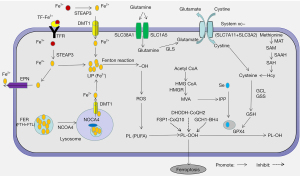
Mounting evidence has implicated ferroptosis in disorders of the cardiovascular, digestive, nervous, and urinary systems, suggesting its potential as a therapeutic target (4-9). In the past decade, a number of specific inducers and inhibitors of ferroptosis have been identified and widely used in the treatment of related diseases (10-15) (Figure 2). Thus, better understanding of the mechanisms of ferroptosis may facilitate the treatment of ferroptosis-related diseases. This article reviews specifically: (I) the origin of ferroptosis; (II) the molecular mechanisms of ferroptosis; (III) the regulation of antioxidant systems in ferroptosis; (IV) the relationship between ferroptosis and diseases. We present the following article in accordance with the Narrative Review reporting checklist (available at https://atm.amegroups.com/article/view/10.21037/atm-21-6942/rc).
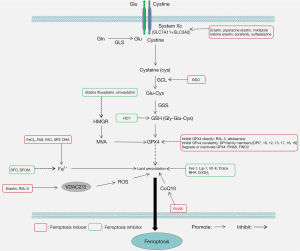
Methods
A literature review was performed using PubMed to search all scientific articles published through to November 2021. The search terms used included: ‘ferroptosis’, ‘ferroptosis inducers’, ‘ferroptosis inhibitors’, ‘ferroptosis and GSH’, ‘ferroptosis and GPX4’, ‘ferroptosis and System Xc-’, ‘SLC7A11’, ‘P53’, ‘NRF2 and ferroptosis’, ‘iron metabolism’, ‘lipid peroxidation’, ‘antioxidant systems’, ‘transsulfuration pathway’, ‘mevalonate pathway’, ‘FSP1-CoQ10’, ‘DHODH-CoQH2’, and ‘GCH1-BH4’. We use a table (Table 1) to present detailed search strategy.
Table 1
Items | Specification |
---|---|
Date of search | 2021.11.30 |
Databases and other sources searched | PubMed |
Search terms used | “ferroptosis”[MeSH] |
“ferroptosis inducers” [MeSH] | |
“ferroptosis inhibitors” [MeSH] | |
(“ferroptosis” [MeSH]) AND “GSH”[MeSH] | |
(“ferroptosis” [MeSH])AND “GPX4” [MeSH] | |
(“ferroptosis” [MeSH]) AND “System Xc-“[MeSH] | |
“SLC7A11” [MeSH] | |
“P53” [MeSH] | |
(“NRF2” [MeSH]) AND “ferroptosis” [MeSH] | |
“iron metabolism” [MeSH] | |
“lipid peroxidation” [MeSH] | |
“antioxidant systems” [MeSH] | |
“transsulfuration pathway” [MeSH] | |
“mevalonate pathway” [MeSH] | |
“FSP1-CoQ10” [MeSH] | |
“DHODH-CoQH2” [MeSH] | |
“GCH1-BH4” [MeSH] | |
Timeframe | 1955–2021 |
Inclusion and exclusion criteria | Focus was placed on original papers and reviews in English about the molecular mechanisms of ferroptosis in antioxidant systems; it excluded articles that have no information about the antioxidant systems and ferroptosis |
Selection process | It was conducted independently by Meng Liu, Xiao-Yu Kong, Yuan Yao; data selection is the intersection of the search of three authors |
Origin of ferroptosis
In 1955, Eagle et al. found that cystine influences mammalian cell proliferation, and in cystine-deficient medium, mouse fibroblasts and human cervical cancer cells underwent a unique form of cell death (16). In the 1970s, Bannai et al. showed that cystine deficiency-induced cell death was accompanied by intracellular GSH reduction and reactive oxygen species (ROS) accumulation, and that the lipophilic antioxidant Vitamin E (Vit-E) reversed cystine deficiency-induced cell death (17). Numerous studies then showed that iron chelating agents also prevented cell death induced by cystine deficiency and GSH depletion, suggesting that this form of cell death is iron-dependent (18-22). Additionally, the small molecular compound erastin and RAS synthetic lethal substance 3 (RSL-3) affected the synthesis of GSH and the ability of GPX4 to induce the above type of cell death (19). In 2012, this unique form of non-apoptotic cell death was named “ferroptosis” (1). In 2018, the cell death nomenclature committee defined ferroptosis as regulatory cell death from intracellular redox imbalance, which can be inhibited by iron chelating agents and lipophilic antioxidants (23).
Mechanisms of ferroptosis
Lipid peroxidation
ROS, mainly produced in mitochondria from normal metabolism and energy production, are essential for cell signaling and tissue homeostasis (24). However, excess ROS have adverse effects on cells such as causing cell damage by attacking various biomolecules. Meanwhile, ROS are detoxified by antioxidants such as GPX, Vit-E, and CoQ10 (25). The imbalance between the generation and elimination rate of ROS leads to various pathologies which are associated with oxidative stress. Among the biomolecules attacked by ROS, lipids are one of the most vulnerable molecules (15). Thus, the level of lipid peroxidation represents the state of oxidative stress.
Lipid peroxidation is a process by which free radical species such as oxygen radicals, peroxyl radicals, and hydroxyl radicals attack the diallylidene of polyunsaturated fatty acids (PUFA), resulting in the accumulation of lipid peroxidation free radicals and hydroperoxide (26). The PUFA content in cells and organelle membranes is high, so lipid peroxidation occurs easily, leading to impaired cell integrity and functions (27). Of note, recent studies have shown that lipid peroxidation is essential for ferroptosis.
Kagan et al. showed that most oxygenated phospholipid species, such as phosphatidylethanolamine-arachidonic acid (PE-AA) and adrenic acid (PE-AdA), are upregulated in ferroptosis induced by GPX4 knockout in vivo and in vitro (28). PE-AA and PE-AdA are important substrates for lipid peroxidation. Previous studies indicated that Acyl-CoA synthetase long-chain family member 4 (ACSL4) and lysophosphatidylcholine acyltransferase 3 (LPCAT3) catalyzed the biosynthesis of PE-AA and PE-AdA (29,30). Consistently, inhibiting lipid peroxidation through the deletion of ACSL4 and LPCAT3 suppressed ferroptosis in cancer cells (31,32). It has been confirmed that the antioxidants Ferrostatin-1 (Fer-1), Liproxstatin-1 (Lip-1), and Vit-E inhibit ferroptosis via protecting cells from lipid peroxides (12,26). The main primary products of lipid peroxidation are lipid hydrogen peroxide (L-OOH) and numerous aldehydes, including malondialdehyde (MDA) and 4-hydroxy-nonenal (4-HNE) (25). MDA and 4-HNE are widely used as ferroptosis markers, although their role in ferroptosis induction needs further investigation. Though the above studies have confirmed the promotive effect of lipid peroxidation on ferroptosis, there is as yet no evidence elucidating the mechanisms of lipid peroxidation-induced ferroptosis.
Iron loading
Lipid peroxidation can be mediated by enzymatic (non-heme iron-containing enzyme lipoxygenase family) and non-enzymatic (iron-catalyzed lipid free radical autoxidation chain reaction, iron-driven oxidative cleavage of hydrogen peroxidation products) processes (26). Both enzymatic and non-enzymatic reactions require iron. Additionally, Fe2+ can directly catalyze H2O2 decomposition into hydroxyl radical through the Fenton reaction, promoting intracellular ROS aggregation and lipid peroxidation (33). Therefore, excess iron (mainly free Fe2+) can directly or indirectly promote lipid peroxidation, suggesting that iron loading is an important mechanism for triggering ferroptosis.
Iron uptake
Uptake of extracellular Fe3+ is mainly mediated by transferrin (TF) and transferrin receptor (TFR). Fe3+-bearing TF binds to TFR on target cells transporting Fe3+ into the cell, where it is reduced to Fe2+ by six-transmembrane epithelial antigen of prostate 3 (STEAP3). The expression of TF and TFR affects cell sensitivity to ferroptosis. TFR knockdown and TF depletion with anti-transferrin antibody in serum could significantly inhibit ferroptosis in mouse embryonic fibroblasts (34). Uptake of extracellular Fe2+ is mediated by divalent metal transporter 1 (DMT1). Song et al. showed that DMT1 overexpression in cardiomyocytes promotes ferroptosis induced by hypoxia/reoxygenation, and increases intracellular ROS accumulation, Fe2+ deposition, and MDA levels (35). In contrast, DMT1 knockout suppressed hypoxia/reoxygenation-induced ferroptosis in cardiomyocytes. In addition, intracellular Fe2+ is secreted to the extracellular space by ferroprotein (FPN). FPN knockout in neuroblastoma cells reduces iron efflux, elevates intracellular iron, and promotes erastin-induced ferroptosis (36).
Iron storage
Most intracellular iron is stored in ferritin (FER), with a small proportion existing in a free state to form the labile iron pool (LIP) (37). FER is a spherical polymer composed of ferritin heavy chain (FTH) and ferritin light chain (FTL) and protects cells from oxidative stress caused by free iron overload (37,38). Autophagic FTH degradation releases Fe2+ which is mediated by nuclear receptor coactivator 4 (NCOA4) (38). Fe2+ is then transported from the lysosome to the cytoplasm by DMT1, elevating intracellular levels of free iron (38). Consistently, inhibition of FER autophagy by ATG5, ATG7, or NCOA4 knockout reduces intracellular free iron and inhibits ferroptosis (39). Numerous studies show that targeting FER can modulate the sensitivity of various cell types to ferroptosis (7,40,41). Targeted knockout of cardiomyocyte FTH induces ferroptosis in mice, leading to mild cardiomyopathy on a normal diet and severe left ventricular (LV) hypertrophy and heart damage on a high-iron diet (7). FTH silencing enhances ferroptosis induction by erastin and sorafenib in hepatoma cells (40). The pentapeptide protein Prominin2 promotes formation of polyvesicular bodies and FER exosomes, reduces intracellular iron levels, and inhibits ferroptosis in breast cancer cells (41). Together, promoting the release of free iron from FER is an important way to trigger ferroptosis.
Iron regulation
Iron regulatory proteins (IRPs), including IRP1 and IRP2, regulate intracellular iron homeostasis. IRPs bind to the iron response element (IRE) in the 5' UTR of iron metabolism-related gene transcripts to regulate their translation. When intracellular free iron is low, IRPs bind to IREs in FER, TFR, and FPN gene transcripts, blocking the translation of FER and FPN and enhancing the translation of TFR, resulting in increased release of free iron, decreased iron efflux, and increased iron uptake (42,43). Dihydroartemisinin (DHA), a semisynthetic derivative of artemisinin, is reported to elevate intracellular free iron through the IRP-IRE pathway, increase the sensitivity of cancer cells to RSL3-induced ferroptosis, and enhance the anticancer effect of GPX4 knockout in mice (44).
Regulation of antioxidant systems in ferroptosis
System Xc-GSH-GPX4 pathway
It is considered that the essence of ferroptosis is the iron-dependent accumulation of lipid hydroperoxides induced by various oxidative stress stimuli, and the System Xc-GSH-GPX4 pathway is the main prevention system for ferroptosis. System Xc- is a cell membrane cystine-glutamate antiporter, comprised of a heterodimer of the light chain subunit SLC7A11 and the heavy chain subunit SLC3A2 (45). System Xc- transports intracellular glutamate to the extracellular space and extracellular cystine into the intracellular space (1). Intracellular cystine is then converted to cysteine, which is combined with glutamic acid and glycine into GSH by glutamate cysteine ligase (GCL) and glutathione synthase (GSS) (18). GPX4 can convert lipid hydrogen peroxide into nontoxic lipid alcohol with GSH as a cofactor, which protects cells from lipid peroxides (46). Thus, when System Xc- is inhibited, GSH synthesis is decreased and GPX4 is inactivated, cell an induce ferroptosis, and targeting this pathway is an important antioxidant system involved in regulating ferroptosis.
System Xc-
Research on ferroptosis regulation by targeting System Xc- activity has mainly focused on the SLC7A11 subunit. In general, various molecules regulate SLC7A11, such as tumor suppressor P53, tumor suppressor BRCA1-associated protein 1 (BAP1), activating transcription factor 3/4 (ATF3/ATF4), beclin 1 (BECN1), and nuclear factor erythroid 2-related factor 2 (NRF2) (Figure 3).
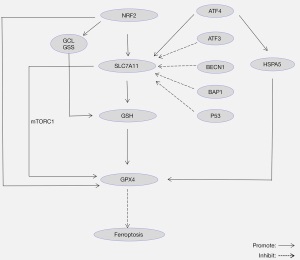
Over the past few years, the role of P53 in ferroptosis has gradually become a burgeoning research direction, and studies have shown that P53 plays a dual role in ferroptosis. On the one hand, P53 suppresses SLC7A11 expression and reduces cystine uptake, triggering ferroptosis (15,47). On the other hand, P53 suppresses erastin-induced ferroptosis by blocking dipeptidyl-peptidase-4 (DPP4) activity in human colorectal cancer (CRC) (48). In addition, Zhang et al. discovered that another tumor suppressor BAP1 promoted ferroptosis and inhibited the growth of cancer cells by inhibiting SLC7A11 (49). The mechanism may be that BAP1 represses the transcription of SLC7A11 by reducing histone 2A ubiquitination (H2Aub) on the SLC7A11 gene (49).
Several transcription factors regulate SLC7A11. ATF3 binds to the SLC7A11 promoter to inhibit the transcription of SLC7A11, thus inhibiting the synthesis of GSH and promoting erastin-induced ferroptosis (50). In contrast, ATF4 binds to the SLC7A11 promoter, activating its expression, thereby inhibiting ferroptosis (50,51). NRF2 is one of the most important transcription factors that regulates ferroptosis. NRF2 is released from the Kelch-like ECH-associated protein 1 (Keap1) binding site and transferred to the nucleus to bind to the antioxidant response elements (AREs) in the target gene promoter region under oxidative stress (52-54). NRF2 binds to the ARE of the SLC7A11 promoter, promoting the synthesis of GSH (52). Dong et al. demonstrated that NRF2 inhibited acute lung injury induced by intestinal ischemia-reperfusion in mice via upregulating the expression of SLC7A11 (55). The target genes of NRF2 also include GPX4, GCL, and GSS (52-54). For example, NRF2 binds to the ARE of the GPX4 promoter, regulating its expression and inhibiting lipid peroxidation (52).
More recently, it was shown that SLC7A11 promoted the synthesis of GPX4 protein to inhibit ferroptosis by activating the mTORC1-4EBP signaling pathway (56). BECN1, a key player in macroautophagy, is reported to directly bind to SLC7A11 and inhibit ferroptosis (57). These two studies suggest that ferroptosis regulated by SLC7A11 may be linked with autophagy. In view of the importance and complexity of SLC7A11 in regulating ferroptosis, it is necessary to understand the regulatory network of SLC7A11 and explore its potential mechanism for finding more targets of ferroptosis.
GSH
GSH, a tripeptide of cysteine, glutamate, and glycine, exists in almost all cells and has important functions such as antioxidation, integration, and detoxification. Previous studies show that silencing GCL, a rate-limiting enzyme in GSH synthesis, is fatal in mice (58,59). A study shows that the GCL inhibitor buthionine sulfoximine (BSO) promotes ferroptosis by suppressing GSH synthesis (60).
Notably, the glutamine metabolism pathway promotes ferroptosis by enhancing glutamine uptake and decomposition of glutamine, elevating intracellular glutamate and decreasing intracellular GSH. Glutamine, which is mediated by SLC38A1 and SLC1A5 receptors on the cell membrane, was shown to degrade to glutamate by glutaminase (GLS) (61). It has been shown that inhibiting glutamine uptake by negative regulation of SLC1A5 using miRNA-137 inhibits lipid peroxidation and iron loading in melanoma cells (62). In a mouse model of myocardial ischemia/reperfusion (I/R) injury, GLS inhibitor compound 968 suppressed ferroptosis by inhibiting glutamine decomposition, thereby reducing LV end-diastolic pressure and inhibiting lactate dehydrogenase (LDH) release during reperfusion (34). Taken together, intracellular glutamate can regulate ferroptosis by affecting the level of GSH.
GPX4
In 2003, Imai et al. showed that GPX4 knockout in mice could lead to early embryonic death (63). In 2008, Seiler et al. showed that conditional GPX4 knockout in mouse primary fibroblasts could lead to massive lipid peroxidation and non-apoptotic cell death (64). Following official recognition of ferroptosis, mounting evidence has shown that GPX4 plays a pivotal role in preventing ferroptosis (51,56,65-67). GPX4 inhibitors, including RSL-3, DPI10, and DPI7, are used to specifically induce ferroptosis (12). In addition, ATF4 is reported to induce binding of heat shock protein family A (Hsp70) member 5 (HSPA5) to GPX4 and regulate ferroptosis in human pancreatic ductal adenocarcinoma cells by inhibiting GPX4 degradation (65).
Transsulfuration pathway
In addition to System Xc-mediated cystine transport into the cell to produce cysteine, cells also produce cysteine through the transsulfuration pathway. This involves the process of transferring sulfur atoms from methionine to serine, thereby producing cysteine. In this way, the transsulfuration pathway is an alternative antioxidant process to inhibit lipid peroxidation when the System Xc-GSH-GPX4 pathway is inhibited.
Methionine is converted to S-adenosyl methionine (SAM) by methionine adenosyl transferase (MAT), which further produces S-adenosylhomocysteine (SAH). SAH is hydrolyzed to homocysteine (HCY), the precursor of cysteine, by S-adenosine homocysteine hydrolase (SAHH) (68,69). Cao et al. reported that high expression of the oxidative stress-related protein DJ-1 stabilizes SAHH activity and promotes HCY synthesis through the transsulfuration pathway (68). Cysteine-tRNA synthetase (CARS), which links cysteine with tRNAs for protein translation, is important in cysteine metabolism (70). Hayano et al. found that CARS knockdown in human and rat fibroma, Ewing’s sarcoma, and osteosarcoma cells upregulates genes related to serine biosynthesis and transsulfuration including PHGDH, PSAT1, and PSPH, and promotes cysteine synthesis, elevates intracellular GSH levels, and inhibits erastin-induced ferroptosis (71).
MVA pathway
The MVA pathway synthesizes isoprene compounds from acetyl-CoA. The process involves the condensation of acetyl-CoA and acetyl coenzyme A to synthesize 3-hydroxy-3-methylglutaryl-CoA (HMG-CoA). HMG-CoA is then converted to MVA by HMG-CoA reductase (HMGR) (72). MVA is further metabolized into isoprene compounds, including cholesterol, isopentenyl pyrophosphate (IPP), and gibberellin A. Past studies have shown that IPP promotes selenocysteine tRNA maturation and selenoprotein GPX4 synthesis, which is vital for the enhancement of GPX4 activity and inhibition of ferroptosis (67,73). Statins suppress HMGR activity and inhibit the MVA pathway, thereby inhibiting GPX4 synthesis (74). Before ferroptosis was recognized, clinical trials had shown that statins are effective in cancer treatment (31). However, there is no evidence that statins affect disease progression by promoting ferroptosis, and further studies are needed to elucidate the relationship between MVA and ferroptosis to determine if statins act by modulating ferroptosis.
FSP1-CoQ10 pathway
Apart from the above GSH/GPX4-dependent pathway, other antioxidant pathways have been reported to inhibit ferroptosis in a GPX4-independent manner. In lung cancer cells, GPX4 inhibition did not induce ferroptosis, indicating the existence of a GSH/GPX4-independent pathway (75,76). Apoptosis-inducing factor mitochondria-associated 2 (AIFM2) is a CoQ10 redox enzyme dependent on nicotinamide-adenine dinucleotide phosphate (NADP) (43). CoQ10 is a lipophilic compound needed by the mitochondrial electron transport chain and lysosomal membranes to produce energy, and can be used as a lipophilic free radical trapping agent. Bersuker et al. showed that AIFM2 (also FSP1) overexpression markedly suppresses ferroptosis caused by GPX4 inhibition and correlates positively with ferroptosis resistance in numerous cancer cell lines (75). The above study suggests that FSP1 can capture lipid free radicals independently of GPX4 and inhibit lipid peroxidation and ferroptosis.
DHODH-CoQH2 pathway
DHODH is a flavin-dependent enzyme in the inner mitochondrial membrane that catalyzes the 4th step of pyrimidine nucleotide synthesis (oxidation of dihydroorotate, DHO, to orotate, OA, and reduction of ubiquinone to dihydroubiquione, CoQH2) (77). CoQH2, which is a radical trapping antioxidant, can inhibit lipid peroxidation in mitochondria. Recently, DHODH has been shown to inhibit mitochondrial ferroptosis by regulating CoQH2 production in the inner mitochondrial membrane (78). DHODH deletion induced lipid peroxidation in cancer cells with low GPX4 expression, and the substrate and product of DHODH could impact the sensitivity of ferroptosis induced by inhibition of GPX4. Thus, the DHODH-CoQH2 pathway is an important antioxidant system to inhibit ferroptosis. This was the first study linking ferroptosis to nucleotide metabolism.
GCH1-BH4 pathway
BH4 is a component of the antioxidant system and is involved in the metabolism of nitric oxide, neurotransmitters, and aromatic amino acids (79). GCH1 is a rate-limiting enzyme in BH4 synthesis (80). Kraft et al. used whole genome library CRISPR/Cas9 screening to show that GCH1 suppresses ferroptosis (81). GCH1 overexpression in mouse fibroblasts significantly inhibited ferroptosis induction by RSL-3 treatment and GPX4 inhibition. Liposome analysis revealed that GCH1 overexpression selectively inhibits peroxidation of some PUFA phospholipids (81). Similarly, GCH1-BH4 pathway regulates ferroptosis by inhibiting lipid peroxidation.
Ferroptosis and disease
Neoplastic disease
Numerous studies have identified ferroptosis as an effective way of killing cancer cells. Mounting evidence suggests that ferroptosis induction enhances sensitivity to anticancer drugs (31,74,82). Ferroptosis induction with erastin and RSL-3 is reported to enhance the anticancer effects of cisplatin and reduce drug resistance to anticancer drugs by inhibiting System Xc- in lung cancer, CRC, ovarian cancer, and pancreatic ductal adenocarcinoma (82).
In recent years, attention has been paid to the role of epigenetic modification in ferroptosis of tumor cell, especially post-translational modification (PTM) of proteins. PTM of proteins (such as phosphorylation, ubiquitin, acetylation and methylation) plays an indispensable role in ferroptosis (43). AMP-activated protein kinase (AMPK) accelerates the phosphorylation of acetyl-CoA carboxylase and inhibits the biosynthesis of PUFA, thus inhibiting ferroptosis in many cancer cell lines (83). According to the latest research, class I histone deacetylase (HDAC) inhibitors enhance ferroptosis in human fibroma HT-1080 cell line (84). Also, tumor suppressor BRCA-1-related protein-1 inhibits the transcription of SLC7A11 by reducing the ubiquitination of histone 2A on SLC7A11 gene, thus triggering ferroptosis (49). One study has been identified that histone H3K9 demethylase KDM3B inhibits erastin-induced ferroptosis by up-regulating the expression of SLC7A11 in conjunction with transcription factor ATF4 in human fibroma HT-1080 cell line (85).
Recent research has sought to uncover ferroptosis-driven anticancer strategies. Tang et al. synthesized manganese-silica nanoparticles (MMSNs) whose oxidation bonds are broken at high GSH levels, causing GSH consumption and triggering ferroptosis in liver cancer cells (86). Ou et al. found that LDL-DHA, which is the low-density lipoprotein nanoparticles reconstituted with docosahexaenoic acid, directly reduces GSH and inactivates GPX4, inducing ferroptosis (87). Additionally, the ferroptosis inducer sorafenib can be encapsulated in a nanostructure composed of Fe3+ and reduced to Fe2+ at tumor-specific sites for the Fenton reaction, resulting in tumor cell-specific ferroptosis (88).
Non-neoplastic disease
Studies have implicated ferroptosis in liver, cardiovascular, kidney, and neurodegenerative disorders (9,89-92). It was reported that RSL-3 increased steatosis, inflammation, and cell damage in mouse livers (90). There is evidence suggesting that excessive ROS production and iron overload promote inflammation, myocardial fibrosis, ventricular remodeling, and pressure overload in mouse hearts (89). A proteomics study of myocardial mouse tissue after myocardial infarction revealed significantly reduced GPX4 protein expression, suggesting that ferroptosis may have occurred in cardiomyocytes (92). Feng et al. found that Lip-1 increased GPX4 levels and reduced ROS levels, reducing the size of myocardial infarction after ischemia and protecting mitochondrial structural integrity (91). Deletion of Panx1, a member of the ATP-release protein family, inhibits lipid peroxidation and iron loading, reducing injury to human kidney HK-2 cells and mouse kidneys (9).
Emerging evidence supports that the inhibition of ferroptosis is an effective measure to reduce ischemia-reperfusion injury (IRI). IRI is a process in which the degree of tissue injury increases rapidly when an organ undergoes a brief decrease or cessation of blood flow and then re-establishes perfusion. A large number of oxygen free radicals can be produced after reperfusion, which is the main pathogenesis of extensive tissue and cell injury. Inhibitor of apoptosis-stimulating protein of P53 (IASPP), which promotes nuclear factor NRF2 accumulation and nuclear translocation, has been proved to inhibit renal and cardiac IRI in vivo and in vitro (93). Similarly, ferroptosis inhibitor Lip-1 has been reported to alleviate intestinal IRI by inhibiting ACSL4 (94). One study has shown that flavonoid Galangin inhibits ferroptosis by activating the SLC7A11/GPX4 axis, thus alleviating cerebral IRI in gerbils (95).
Iron accumulation and lipid peroxidation are important factors in the pathogenesis of neurodegenerative diseases. It is well documented that ferroptosis promotes the occurrence and development of neurodegenerative diseases such as Alzheimer’s, Parkinson’s, and Huntington’s disease (4). Early clinical trials involving Parkinson’s disease patients found that N-acetylcysteine, a precursor of GSH synthesis, improves motor symptoms (8). Together, inhibition of ferroptosis may be a preventive measure for non-neoplastic diseases in general.
In addition, previous studies have shown that inhibition of apoptosis promotes cell reprogramming, which is of great significance to reverse aging (96-98). In pararal, current studies have demonstrated that inhibition of ferroptosis can effectively promote cell reprogramming, though the molecular mechanisms of phospholipid oxidation signal transduction are different from apoptosis (97,99). The oxidation of polyunsaturated phospholipids leads to a large number of oxidation products, which have different chemical structures and play different biological effects on the membrane (100). In apoptosis, it is mainly phosphatidyl serine oxidation, which initiates the inherent apoptotic cascade reaction (100). In ferroptosis, phosphatidylethanolamines (PEs), specifically arachidonic acid (AA) and adrenic acid (ADA) containing PEs, is prone to oxidation and induce ferroptosis (24). Ferroptosis inhibitors, including Lip-1 and Vit-E, can effectively increase neuronal reprogramming by inhibiting lipid peroxidation (97).
Conclusions and prospect
Lipid peroxidation is central to ferroptosis, and is also a hub for antioxidant systems. Lipid peroxidation products directly damage cell and organelle membranes, causing cell death. However, few studies have investigated whether the mechanisms underlying ferroptosis in various cellular organelles are the same. Because iron loading promotes ferroptosis, the therapeutic effect of iron chelators against ferroptosis-related diseases has been widely demonstrated in cell and animal models (4,45). However, a recent study has shown that copper may induce glutamate-induced oxidative damage and cell death in HT22 cells by reducing the activity of GCL, a rate-limiting enzyme in GSH synthesis, indicating that other metal ions may influence ferroptosis (101).
Ferroptosis is a form of cell death that is associated with iron, lipid, amino acid, and pyrimidine nucleotide metabolism. Lee et al. showed that energy stress is also associated with ferroptosis and that glucose starvation inhibited ferroptosis induction by erastin in mouse fibroblasts, partly through AMP-activated protein kinase (AMPK) activation, which in turn inactivates acetyl-CoA-carboxylase (ACC), suppressing PUFA biosynthesis (83). Their research differs from previous studies in two ways: first, glucose starvation alone induces ROS production and apoptosis, and second, another study found that AMPK-mediated BECN1 phosphorylation promotes ferroptosis in numerous cancer cells by directly blocking System Xc- activity (57). Thus, the molecular basis of ferroptosis may differ by cell type and stress stimuli.
As mentioned above, ferroptosis promotes the clearance of cancer cells and its inducers increase the sensitivity of many anticancer drugs. In order to treat cancer more effectively and economically, ferroptosis inducers can be used alone or in combination with chemotherapeutic drugs to increase the therapeutic effect of cancer. Moreover, it’s an excellent practice to improve the delivery mode of traditional anticancer drugs, such as adding anticancer drugs to nanoparticles that induces ferroptosis specifically. There has been growing interest in the relationship between ferroptosis and various diseases, but there are few studies on the downstream effects of ferroptosis; that is, how it triggers disease occurrence and progression. Hence, it is crucial to elucidate the mechanisms of ferroptosis in different diseases. Future studies should investigate the basis of ferroptosis and its relationship with other forms of cell death in dynamic environments. This would improve our understanding of the occurrence, progression, diagnosis, and treatment of ferroptosis-related disorders.
The molecular mechanisms and therapeutic strategies of oxidative stress-induced ferroptosis in tumor cells have been reported in the past (102), but this article more comprehensively summarizes the molecular mechanisms of ferroptosis in various cells. It focuses on the relationship between antioxidant systems and ferroptosis, reveals the inhibitory role of antioxidant system in ferroptosis, and provides new ideas for the treatment of ferrroptosis-related diseases (neoplastic diseases and non-neoplastic diseases).
Acknowledgments
Funding: This work was supported by the Medical and Health Research Project of Yichang City (No. A21-2-010).
Footnote
Reporting Checklist: The authors have completed the Narrative Review reporting checklist. Available at https://atm.amegroups.com/article/view/10.21037/atm-21-6942/rc
Conflicts of Interest: All authors have completed the ICMJE uniform disclosure form (available at https://atm.amegroups.com/article/view/10.21037/atm-21-6942/coif). The authors have no conflicts of interest to declare.
Ethical Statement: The authors are accountable for all aspects of the work in ensuring that questions related to the accuracy or integrity of any part of the work are appropriately investigated and resolved.
Open Access Statement: This is an Open Access article distributed in accordance with the Creative Commons Attribution-NonCommercial-NoDerivs 4.0 International License (CC BY-NC-ND 4.0), which permits the non-commercial replication and distribution of the article with the strict proviso that no changes or edits are made and the original work is properly cited (including links to both the formal publication through the relevant DOI and the license). See: https://creativecommons.org/licenses/by-nc-nd/4.0/.
References
- Dixon SJ, Lemberg KM, Lamprecht MR, et al. Ferroptosis: an iron-dependent form of nonapoptotic cell death. Cell 2012;149:1060-72. [Crossref] [PubMed]
- Tang D, Chen X, Kang R, et al. Ferroptosis: molecular mechanisms and health implications. Cell Res 2021;31:107-25. [Crossref] [PubMed]
- Jiang M, Hu R, Yu R, et al. A narrative review of mechanisms of ferroptosis in cancer: new challenges and opportunities. Ann Transl Med 2021;9:1599. [Crossref] [PubMed]
- Ratan RR. The Chemical Biology of Ferroptosis in the Central Nervous System. Cell Chem Biol 2020;27:479-98. [Crossref] [PubMed]
- Mao L, Zhao T, Song Y, et al. The emerging role of ferroptosis in non-cancer liver diseases: hype or increasing hope? Cell Death Dis 2020;11:518. [Crossref] [PubMed]
- Lu D, Xia Q, Yang Z, et al. ENO3 promoted the progression of NASH by negatively regulating ferroptosis via elevation of GPX4 expression and lipid accumulation. Ann Transl Med 2021;9:661. [Crossref] [PubMed]
- Fang X, Cai Z, Wang H, et al. Loss of Cardiac Ferritin H Facilitates Cardiomyopathy via Slc7a11-Mediated Ferroptosis. Circ Res 2020;127:486-501. [Crossref] [PubMed]
- Monti DA, Zabrecky G, Kremens D, et al. N-Acetyl Cysteine May Support Dopamine Neurons in Parkinson's Disease: Preliminary Clinical and Cell Line Data. PLoS One 2016;11:e0157602. [Crossref] [PubMed]
- Su L, Jiang X, Yang C, et al. Pannexin 1 mediates ferroptosis that contributes to renal ischemia/reperfusion injury. J Biol Chem 2019;294:19395-404. [Crossref] [PubMed]
- Stockwell BR, Jiang X, Gu W. Emerging Mechanisms and Disease Relevance of Ferroptosis. Trends Cell Biol 2020;30:478-90. [Crossref] [PubMed]
- Chen X, Li J, Kang R, et al. Ferroptosis: machinery and regulation. Autophagy 2021;17:2054-81. [Crossref] [PubMed]
- Li J, Cao F, Yin HL, et al. Ferroptosis: past, present and future. Cell Death Dis 2020;11:88. [Crossref] [PubMed]
- Xie Y, Hou W, Song X, et al. Ferroptosis: process and function. Cell Death Differ 2016;23:369-79. [Crossref] [PubMed]
- Cao JY, Dixon SJ. Mechanisms of ferroptosis. Cell Mol Life Sci 2016;73:2195-209. [Crossref] [PubMed]
- Liang C, Zhang X, Yang M, et al. Recent Progress in Ferroptosis Inducers for Cancer Therapy. Adv Mater 2019;31:e1904197. [Crossref] [PubMed]
- Eagle H. Nutrition needs of mammalian cells in tissue culture. Science 1955;122:501-14. [Crossref] [PubMed]
- Bannai S, Tsukeda H, Okumura H. Effect of antioxidants on cultured human diploid fibroblasts exposed to cystine-free medium. Biochem Biophys Res Commun 1977;74:1582-8. [Crossref] [PubMed]
- Bayır H, Anthonymuthu TS, Tyurina YY, et al. Achieving Life through Death: Redox Biology of Lipid Peroxidation in Ferroptosis. Cell Chem Biol 2020;27:387-408. [Crossref] [PubMed]
- Hirschhorn T, Stockwell BR. The development of the concept of ferroptosis. Free Radic Biol Med 2019;133:130-43. [Crossref] [PubMed]
- Rosin C, Bates TE, Skaper SD. Excitatory amino acid induced oligodendrocyte cell death in vitro: receptor-dependent and -independent mechanisms. J Neurochem 2004;90:1173-85. [Crossref] [PubMed]
- Murphy TH, Miyamoto M, Sastre A, et al. Glutamate toxicity in a neuronal cell line involves inhibition of cystine transport leading to oxidative stress. Neuron 1989;2:1547-58. [Crossref] [PubMed]
- Murphy TH, Schnaar RL, Coyle JT. Immature cortical neurons are uniquely sensitive to glutamate toxicity by inhibition of cystine uptake. FASEB J 1990;4:1624-33. [Crossref] [PubMed]
- Galluzzi L, Vitale I, Aaronson SA, et al. Molecular mechanisms of cell death: recommendations of the Nomenclature Committee on Cell Death 2018. Cell Death Differ 2018;25:486-541. [Crossref] [PubMed]
- Latunde-Dada GO. Ferroptosis: Role of lipid peroxidation, iron and ferritinophagy. Biochim Biophys Acta Gen Subj 2017;1861:1893-900. [Crossref] [PubMed]
- Su LJ, Zhang JH, Gomez H, et al. Reactive Oxygen Species-Induced Lipid Peroxidation in Apoptosis, Autophagy, and Ferroptosis. Oxid Med Cell Longev 2019;2019:5080843. [Crossref] [PubMed]
- Kajarabille N, Latunde-Dada GO. Programmed Cell-Death by Ferroptosis: Antioxidants as Mitigators. Int J Mol Sci 2019;20:4968. [Crossref] [PubMed]
- Gaschler MM, Stockwell BR. Lipid peroxidation in cell death. Biochem Biophys Res Commun 2017;482:419-25. [Crossref] [PubMed]
- Kagan VE, Mao G, Qu F, et al. Oxidized arachidonic and adrenic PEs navigate cells to ferroptosis. Nat Chem Biol 2017;13:81-90. [Crossref] [PubMed]
- Shindou H, Shimizu T. Acyl-CoA:lysophospholipid acyltransferases. J Biol Chem 2009;284:1-5. [Crossref] [PubMed]
- Soupene E, Kuypers FA. Mammalian long-chain acyl-CoA synthetases. Exp Biol Med (Maywood) 2008;233:507-21. [Crossref] [PubMed]
- Chen X, Kang R, Kroemer G, et al. Broadening horizons: the role of ferroptosis in cancer. Nat Rev Clin Oncol 2021;18:280-96. [Crossref] [PubMed]
- Dixon SJ, Winter GE, Musavi LS, et al. Human Haploid Cell Genetics Reveals Roles for Lipid Metabolism Genes in Nonapoptotic Cell Death. ACS Chem Biol 2015;10:1604-9. [Crossref] [PubMed]
- Wardman P, Candeias LP. Fenton chemistry: an introduction. Radiat Res 1996;145:523-31. [Crossref] [PubMed]
- Gao M, Monian P, Quadri N, et al. Glutaminolysis and Transferrin Regulate Ferroptosis. Mol Cell 2015;59:298-308. [Crossref] [PubMed]
- Song Y, Wang B, Zhu X, et al. Human umbilical cord blood-derived MSCs exosome attenuate myocardial injury by inhibiting ferroptosis in acute myocardial infarction mice. Cell Biol Toxicol 2021;37:51-64. [Crossref] [PubMed]
- Geng N, Shi BJ, Li SL, et al. Knockdown of ferroportin accelerates erastin-induced ferroptosis in neuroblastoma cells. Eur Rev Med Pharmacol Sci 2018;22:3826-36. [PubMed]
- Theil EC. Ferritin: structure, gene regulation, and cellular function in animals, plants, and microorganisms. Annu Rev Biochem 1987;56:289-315. [Crossref] [PubMed]
- Fuhrmann DC, Mondorf A, Beifuß J, et al. Hypoxia inhibits ferritinophagy, increases mitochondrial ferritin, and protects from ferroptosis. Redox Biol 2020;36:101670. [Crossref] [PubMed]
- Hou W, Xie Y, Song X, et al. Autophagy promotes ferroptosis by degradation of ferritin. Autophagy 2016;12:1425-8. [Crossref] [PubMed]
- Sun X, Ou Z, Chen R, et al. Activation of the p62-Keap1-NRF2 pathway protects against ferroptosis in hepatocellular carcinoma cells. Hepatology 2016;63:173-84. [Crossref] [PubMed]
- Brown CW, Amante JJ, Chhoy P, et al. Prominin2 Drives Ferroptosis Resistance by Stimulating Iron Export. Dev Cell 2019;51:575-86.e4. [Crossref] [PubMed]
- Huang BW, Miyazawa M, Tsuji Y. Distinct regulatory mechanisms of the human ferritin gene by hypoxia and hypoxia mimetic cobalt chloride at the transcriptional and post-transcriptional levels. Cell Signal 2014;26:2702-9. [Crossref] [PubMed]
- Wei X, Yi X, Zhu XH, et al. Posttranslational Modifications in Ferroptosis. Oxid Med Cell Longev 2020;2020:8832043. [Crossref] [PubMed]
- Chen GQ, Benthani FA, Wu J, et al. Artemisinin compounds sensitize cancer cells to ferroptosis by regulating iron homeostasis. Cell Death Differ 2020;27:242-54. [Crossref] [PubMed]
- Sato H, Tamba M, Kuriyama-Matsumura K, et al. Molecular cloning and expression of human xCT, the light chain of amino acid transport system xc-. Antioxid Redox Signal 2000;2:665-71. [Crossref] [PubMed]
- Yang WS, Kim KJ, Gaschler MM, et al. Peroxidation of polyunsaturated fatty acids by lipoxygenases drives ferroptosis. Proc Natl Acad Sci U S A 2016;113:E4966-75. [Crossref] [PubMed]
- Kang R, Kroemer G, Tang D. The tumor suppressor protein p53 and the ferroptosis network. Free Radic Biol Med 2019;133:162-8. [Crossref] [PubMed]
- Xie Y, Zhu S, Song X, et al. The Tumor Suppressor p53 Limits Ferroptosis by Blocking DPP4 Activity. Cell Rep 2017;20:1692-704. [Crossref] [PubMed]
- Zhang Y, Koppula P, Gan B. Regulation of H2A ubiquitination and SLC7A11 expression by BAP1 and PRC1. Cell Cycle 2019;18:773-83. [Crossref] [PubMed]
- Wang L, Liu Y, Du T, et al. ATF3 promotes erastin-induced ferroptosis by suppressing system Xc. Cell Death Differ 2020;27:662-75. [Crossref] [PubMed]
- Chen D, Fan Z, Rauh M, et al. ATF4 promotes angiogenesis and neuronal cell death and confers ferroptosis in a xCT-dependent manner. Oncogene 2017;36:5593-608. [Crossref] [PubMed]
- Dodson M, Castro-Portuguez R, Zhang DD. NRF2 plays a critical role in mitigating lipid peroxidation and ferroptosis. Redox Biol 2019;23:101107. [Crossref] [PubMed]
- Fan Z, Wirth AK, Chen D, et al. Nrf2-Keap1 pathway promotes cell proliferation and diminishes ferroptosis. Oncogenesis 2017;6:e371. [Crossref] [PubMed]
- Abdalkader M, Lampinen R, Kanninen KM, et al. Targeting Nrf2 to Suppress Ferroptosis and Mitochondrial Dysfunction in Neurodegeneration. Front Neurosci 2018;12:466. [Crossref] [PubMed]
- Dong H, Qiang Z, Chai D, et al. Nrf2 inhibits ferroptosis and protects against acute lung injury due to intestinal ischemia reperfusion via regulating SLC7A11 and HO-1. Aging (Albany NY) 2020;12:12943-59. [Crossref] [PubMed]
- Zhang Y, Swanda RV, Nie L, et al. mTORC1 couples cyst(e)ine availability with GPX4 protein synthesis and ferroptosis regulation. Nat Commun 2021;12:1589. [Crossref] [PubMed]
- Song X, Zhu S, Chen P, et al. AMPK-Mediated BECN1 Phosphorylation Promotes Ferroptosis by Directly Blocking System Xc- Activity. Curr Biol 2018;28:2388-2399.e5. [Crossref] [PubMed]
- Dalton TP, Chen Y, Schneider SN, et al. Genetically altered mice to evaluate glutathione homeostasis in health and disease. Free Radic Biol Med 2004;37:1511-26. [Crossref] [PubMed]
- Shi ZZ, Osei-Frimpong J, Kala G, et al. Glutathione synthesis is essential for mouse development but not for cell growth in culture. Proc Natl Acad Sci U S A 2000;97:5101-6. [Crossref] [PubMed]
- Nishizawa S, Araki H, Ishikawa Y, et al. Low tumor glutathione level as a sensitivity marker for glutamate-cysteine ligase inhibitors. Oncol Lett 2018;15:8735-43. [Crossref] [PubMed]
- McGivan JD, Bungard CI. The transport of glutamine into mammalian cells. Front Biosci 2007;12:874-82. [Crossref] [PubMed]
- Luo M, Wu L, Zhang K, et al. miR-137 regulates ferroptosis by targeting glutamine transporter SLC1A5 in melanoma. Cell Death Differ 2018;25:1457-72. [Crossref] [PubMed]
- Imai H, Nakagawa Y. Biological significance of phospholipid hydroperoxide glutathione peroxidase (PHGPx, GPx4) in mammalian cells. Free Radic Biol Med 2003;34:145-69. [Crossref] [PubMed]
- Seiler A, Schneider M, Förster H, et al. Glutathione peroxidase 4 senses and translates oxidative stress into 12/15-lipoxygenase dependent- and AIF-mediated cell death. Cell Metab 2008;8:237-48. [Crossref] [PubMed]
- Zhu S, Zhang Q, Sun X, et al. HSPA5 Regulates Ferroptotic Cell Death in Cancer Cells. Cancer Res 2017;77:2064-77. [Crossref] [PubMed]
- Ursini F, Maiorino M. Lipid peroxidation and ferroptosis: The role of GSH and GPx4. Free Radic Biol Med 2020;152:175-85. [Crossref] [PubMed]
- Ingold I, Berndt C, Schmitt S, et al. Selenium Utilization by GPX4 Is Required to Prevent Hydroperoxide-Induced Ferroptosis. Cell 2018;172:409-22.e21. [Crossref] [PubMed]
- Cao J, Chen X, Jiang L, et al. DJ-1 suppresses ferroptosis through preserving the activity of S-adenosyl homocysteine hydrolase. Nat Commun 2020;11:1251. [Crossref] [PubMed]
- Chen H, Liu S, Ji L, et al. Folic Acid Supplementation Mitigates Alzheimer's Disease by Reducing Inflammation: A Randomized Controlled Trial. Mediators Inflamm 2016;2016:5912146. [Crossref] [PubMed]
- Yao P, Fox PL. Aminoacyl-tRNA synthetases in medicine and disease. EMBO Mol Med 2013;5:332-43. [Crossref] [PubMed]
- Hayano M, Yang WS, Corn CK, et al. Loss of cysteinyl-tRNA synthetase (CARS) induces the transsulfuration pathway and inhibits ferroptosis induced by cystine deprivation. Cell Death Differ 2016;23:270-8. [Crossref] [PubMed]
- Liao P, Hemmerlin A, Bach TJ, et al. The potential of the mevalonate pathway for enhanced isoprenoid production. Biotechnol Adv 2016;34:697-713. [Crossref] [PubMed]
- Warner GJ, Berry MJ, Moustafa ME, et al. Inhibition of selenoprotein synthesis by selenocysteine tRNASerSec lacking isopentenyladenosine. J Biol Chem 2000;275:28110-9. [Crossref] [PubMed]
- Yu H, Guo P, Xie X, et al. Ferroptosis, a new form of cell death, and its relationships with tumourous diseases. J Cell Mol Med 2017;21:648-57. [Crossref] [PubMed]
- Bersuker K, Hendricks JM, Li Z, et al. The CoQ oxidoreductase FSP1 acts parallel to GPX4 to inhibit ferroptosis. Nature 2019;575:688-92. [Crossref] [PubMed]
- Stockwell BR, Friedmann Angeli JP, Bayir H, et al. Ferroptosis: A Regulated Cell Death Nexus Linking Metabolism, Redox Biology, and Disease. Cell 2017;171:273-85. [Crossref] [PubMed]
- Vyas VK, Ghate M. CoMFA and CoMSIA studies on aryl carboxylic acid amide derivatives as dihydroorotate dehydrogenase (DHODH) inhibitors. Curr Comput Aided Drug Des 2012;8:271-82. [Crossref] [PubMed]
- Mao C, Liu X, Zhang Y, et al. DHODH-mediated ferroptosis defence is a targetable vulnerability in cancer. Nature 2021;593:586-90. [Crossref] [PubMed]
- Cronin SJF, Seehus C, Weidinger A, et al. The metabolite BH4 controls T cell proliferation in autoimmunity and cancer. Nature 2018;563:564-8. [Crossref] [PubMed]
- Latremoliere A, Costigan M. GCH1, BH4 and pain. Curr Pharm Biotechnol 2011;12:1728-41. [Crossref] [PubMed]
- Kraft VAN, Bezjian CT, Pfeiffer S, et al. GTP Cyclohydrolase 1/Tetrahydrobiopterin Counteract Ferroptosis through Lipid Remodeling. ACS Cent Sci 2020;6:41-53. [Crossref] [PubMed]
- Wu Y, Yu C, Luo M, et al. Ferroptosis in Cancer Treatment: Another Way to Rome. Front Oncol 2020;10:571127. [Crossref] [PubMed]
- Lee H, Zandkarimi F, Zhang Y, et al. Energy-stress-mediated AMPK activation inhibits ferroptosis. Nat Cell Biol 2020;22:225-34. [Crossref] [PubMed]
- Zille M, Kumar A, Kundu N, et al. Ferroptosis in Neurons and Cancer Cells Is Similar But Differentially Regulated by Histone Deacetylase Inhibitors. eNeuro 2019;6. eENEURO. [Crossref] [PubMed]
- Wang Y, Zhao Y, Wang H, et al. Histone demethylase KDM3B protects against ferroptosis by upregulating SLC7A11. FEBS Open Bio 2020;10:637-43. [Crossref] [PubMed]
- Tang H, Chen D, Li C, et al. Dual GSH-exhausting sorafenib loaded manganese-silica nanodrugs for inducing the ferroptosis of hepatocellular carcinoma cells. Int J Pharm 2019;572:118782. [Crossref] [PubMed]
- Ou W, Mulik RS, Anwar A, et al. Low-density lipoprotein docosahexaenoic acid nanoparticles induce ferroptotic cell death in hepatocellular carcinoma. Free Radic Biol Med 2017;112:597-607. [Crossref] [PubMed]
- Liu T, Liu W, Zhang M, et al. Ferrous-Supply-Regeneration Nanoengineering for Cancer-Cell-Specific Ferroptosis in Combination with Imaging-Guided Photodynamic Therapy. ACS Nano 2018;12:12181-92. [Crossref] [PubMed]
- Jackson AO, Rahman GA, Long S. Attenuating senescence and dead cells accumulation as heart failure therapy: Break the communication networks. Int J Cardiol 2021;334:72-85. [Crossref] [PubMed]
- Qi J, Kim JW, Zhou Z, et al. Ferroptosis Affects the Progression of Nonalcoholic Steatohepatitis via the Modulation of Lipid Peroxidation-Mediated Cell Death in Mice. Am J Pathol 2020;190:68-81. [Crossref] [PubMed]
- Feng Y, Madungwe NB, Imam Aliagan AD, et al. Liproxstatin-1 protects the mouse myocardium against ischemia/reperfusion injury by decreasing VDAC1 levels and restoring GPX4 levels. Biochem Biophys Res Commun 2019;520:606-11. [Crossref] [PubMed]
- Park TJ, Park JH, Lee GS, et al. Quantitative proteomic analyses reveal that GPX4 downregulation during myocardial infarction contributes to ferroptosis in cardiomyocytes. Cell Death Dis 2019;10:835. [Crossref] [PubMed]
- Li Y, Cao Y, Xiao J, et al. Inhibitor of apoptosis-stimulating protein of p53 inhibits ferroptosis and alleviates intestinal ischemia/reperfusion-induced acute lung injury. Cell Death Differ 2020;27:2635-50. [Crossref] [PubMed]
- Li Y, Feng D, Wang Z, et al. Ischemia-induced ACSL4 activation contributes to ferroptosis-mediated tissue injury in intestinal ischemia/reperfusion. Cell Death Differ 2019;26:2284-99. [Crossref] [PubMed]
- Guan X, Li Z, Zhu S, et al. Galangin attenuated cerebral ischemia-reperfusion injury by inhibition of ferroptosis through activating the SLC7A11/GPX4 axis in gerbils. Life Sci 2021;264:118660. [Crossref] [PubMed]
- Kim EJY, Anko ML, Flensberg C, et al. BAK/BAX-Mediated Apoptosis Is a Myc-Induced Roadblock to Reprogramming. Stem Cell Reports 2018;10:331-8. [Crossref] [PubMed]
- Gascón S, Murenu E, Masserdotti G, et al. Identification and Successful Negotiation of a Metabolic Checkpoint in Direct Neuronal Reprogramming. Cell Stem Cell 2016;18:396-409. [Crossref] [PubMed]
- Wang Y, Chen S, Jiang Q, et al. TFAP2C facilitates somatic cell reprogramming by inhibiting c-Myc-dependent apoptosis and promoting mesenchymal-to-epithelial transition. Cell Death Dis 2020;11:482. [Crossref] [PubMed]
- Huang L, McClatchy DB, Maher P, et al. Intracellular amyloid toxicity induces oxytosis/ferroptosis regulated cell death. Cell Death Dis 2020;11:828. [Crossref] [PubMed]
- Fruhwirth GO, Hermetter A. Mediation of apoptosis by oxidized phospholipids. Subcell Biochem 2008;49:351-67. [Crossref] [PubMed]
- Maher P. Potentiation of glutathione loss and nerve cell death by the transition metals iron and copper: Implications for age-related neurodegenerative diseases. Free Radic Biol Med 2018;115:92-104. [Crossref] [PubMed]
- Zhu J, Xiong Y, Zhang Y, et al. The Molecular Mechanisms of Regulating Oxidative Stress-Induced Ferroptosis and Therapeutic Strategy in Tumors. Oxid Med Cell Longev 2020;2020:8810785. [Crossref] [PubMed]
(English Language Editor: C. Betlazar-Maseh)