Comparison of 3D printed anatomical model qualities in acetabular fracture representation
Introduction
Acetabular fracture is defined as damage to the pelvic bone at the hip joint, and accounts for roughly 10% of the 300,000 hip fractures that occur in the US every year; with an average 37 per 100,000 of these causing damage to the acetabulum as well (1,2). Repair of the pelvic bone can require complex orthopedic intervention at the acetabulum and on occasion the femur as well. These types of acetabular injuries are often caused by one of two issues: high-impact incidents such as falls from large heights or vehicle accidents, or low-impact incidents alongside bone degradation due to age or other comorbidity. The necessity for surgical intervention has been most commonly used in high-impact incidents (such as motor incidents), which have a higher occurrence in developing countries due to things such as industrialization, less access to healthcare, and an increased number of motor vehicles per capita (2,3).
In regard to acetabular repair, less developed countries with limited resources face a number of barriers. The incidence of high-impact fractures is much higher and continuing to increase compared to more developed countries, which may be a result of industrialization (2). Additionally, the number of facilities with trained individuals is significantly less than those in developed countries. Previous studies have reported that while physicians in developing countries are generally trained in orthopedics, a majority of them have never performed an acetabular fracture repair (4). While the outcomes of many of lower-limb orthopedic intra-articular operations are defined as satisfactory, a reported 33% of acetabular repairs are defined as “fair or poor” and require either follow up visits or additional operations (5). Additional needs for these countries identified by Whiting et al. included things such as resources, equipment, and post-operation therapy to help improve the outcomes of acetabular surgery (4).
One such method that has been used to improve the outcome of surgical interventions is the usage of 3D printed anatomical models. These models have been used to assist with complex operations ranging from tumor resection within the heart and spine (6,7), and have proven to be especially useful in the field of orthopedics (8,9). Previous studies have found improvements in surgical planning due to increased spatial understanding of the region of interest when using a 3D printed anatomical model (10). This could help to improve surgical outcomes at institutions with less resources, including developing nations. However, many of the models developed for these purposes utilize high-end printers and materials that are not accessible to facilities with less resources. While some models for surgical planning have been developed using low-cost equipment, their accuracy may be of question. Some reports have found significant differences between models developed using different methods (11), which may discourage some hospitals from using 3D printed anatomical models as a part of their surgical planning despite its reported benefits for surgical planning.
The purpose of this study was to develop anatomical models specifically for acetabular repair using previously described methods, as well as low-cost alternatives and to compare the accuracy of the different development methods. The researchers hypothesized that there would be no significant difference between the development methods and that any method of anatomic model development would be beneficial for surgical planning. We present the following article in accordance with the MDAR reporting checklist (available at https://atm.amegroups.com/article/view/10.21037/atm-21-5069/rc).
Methods
This study was approved through the IRB at the University of Nebraska Medical Center (258-18-EP) with waiver of consent due to the data being deidentified and accessed retrospectively. Five acetabular fractures, including the entire pelvis, were modeled using two approaches and printed on four 3D printers (total of 20 models). Models were created from the same CT scan using two different segmentation software packages and then 3D printed on a variety of “high-end” and “low-cost” printers to assess differences between the production methods (Figure 1). The study was conducted in accordance with the Declaration of Helsinki (as revised in 2013).
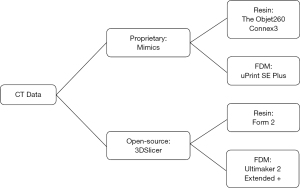
Experimental procedure
To assess the printed models’ accuracy in replicating the correct size of the patient’s pelvic bone, the distance from the posterior inferior iliac spine to the ischial spine was recorded from the CT scan and compared to physical measurements taken directly on the 3D printed models. A unique fracture fragment was also measured directly on the CT imaging data and then compared to physical measurements taken from the printed models. To assess the overall quality of the 3D printed models, they were given to 5 practicing physicians (MD) who completed a survey for each model. The survey data was analyzed to observe differences in representation and their potential utility within the operating room.
Model development
The CT scans utilized were acquired from five CT pelvis studies, with slice thicknesses of 1.25 mm for 4 models and 0.625 mm for 1 model. In-plane pixel resolution ranged from 0.6–0.8 mm. Models were segmented using semi-automatic thresholding techniques on both Mimics (Version 20.0, Materialise, Belgium; cost $12,975.00) and 3D Slicer (Version 4.8.1, Brigham and Women’s Hospital, Boston, MA; cost free, open-source) (12-14). Segmentation was performed using a combination of semi-automatic and manual thresholding techniques by experienced medical image segmentation specialists and verified by a licensed radiologist. Each segmentation on both 3D Slicer and Mimics took a similar amount of time, at approximately 60 minutes per model. To standardize the orientation of each of the printing methods, all models were printed with the acetabulum facing upward on the build platform. Post-processing procedures varied per printer: Objet model support was removed via a high-pressure water cleaner (Powerblast, Balco, UK), uPrint support was removed via a dissolvable support solution (ABS, Stratasys, Inc. Eden Praire, MN, USA), Form 2 support was removed manually and soaked in an isopropyl alcohol solvent bath, and Ultimaker support was removed manually.
Model grouping
Models were developed using two different methods: a high-cost method that involved proprietary segmentation software and high-quality 3D printers, and a low-cost method that utilized open-source segmentation software and low-cost desktop 3D printers to account for the varying level of resources that different institutions may have. The models segmented using the proprietary software Mimics were printed on two high-end printers and focused on two popular methods of printing: resin-based SLA printing and hard-plastic filament based FDM printing. The printers used in this study include the Objet260 Connex3 (Stratasys, Inc. Eden Prairie, MN; cost $158,900.00) which is a high-end 3D resin-based printer that is capable of printing with multiple colors and materials, and the uPrint SE Plus (Stratasys, Inc. Eden Prairie, MN; cost $26,934.00), a high-end industrial FDM printer with dissolvable support structures. The models were also segmented using open-source software 3DSlicer and were printed on two low-cost desktop 3D printers: the Form 2 (Formlabs, Somerville, MA; cost $3,350.00), a stereolithography (SLA) printer that uses liquid resin, and the Ultimaker 2 Extended + (Ultimaker B.V., Geldermalsen, The Netherlands; cost $2,999.00), an FDM printer without dissolvable supports. An outline of the segmentation software and printers used can be found in Figure 1.
Model price
The price to produce each model was based solely on the amount of material used and did not include engineering time, since roughly each model took approximately 60 minutes to develop (segmentation time). The price for each uPrint (high-end FDM) model was $43.04±6.80 and $33.64±5.52 for each Form 2 (low-cost resin) model. The price per Objet (high-end resin) model was significantly higher at $107.70±9.82, and the price of the Ultimaker (low-cost FDM) model was significantly lower at $4.00±0.69 per model. Additional information regarding printing time can be found on Table 1.
Table 1
Printer | Printing time (hh:mm) | Material cost ($) | Measurement 1 accuracy error (mm) | Measurement 2 accuracy error (mm) |
---|---|---|---|---|
Form 2 | 15 h 20 m ± 2 h 07 m | $33.64±$5.52 | 0.44±0.48 | 0.30±0.12 |
Objet | 20 h 03 m ± 2 h 23 m | $107.70±$9.82 | 0.28±0.35 | 0.04±0.09 |
Ultimaker | 16 h 40 m ± 3 h 23 m | $4.00±$0.69 | 0.82±0.47 | 0.34±0.17 |
uPrint | 29 h 04 m ± 3 h 56 m | $43.04±$6.80 | 0.30±0.12 | 0.30±0.12 |
Print times are as reported by the printer. Costs are calculated in USD ($). Measurement 1 Accuracy Error is the mean absolute error between the posterior inferior iliac spine and the ischial spine (as measured in mm) and Measurement 2 Accuracy Error is the mean absolute error for the fracture fragment (as measured in mm).
Model evaluation
The 3D printed models were evaluated based on their accuracy as well as their utility in displaying the clinically significant information. In order to measure model accuracy in displaying the region of interest, digital measurements were taken on the original CT imaging data using the Change picture archiving and communication system (PACS) (Change Healthcare, USA) of the distance between specific landmarks and then replicated on the physical 3D printed models. Measurements were performed on multiplanar maximum intensity projection (MIP) 10 mm reformats, which were sufficient to identify the landmarks of interest. The distance between the posterior inferior iliac spine (PIIS) and the ischial spine was measured across all models as an easily identifiable anatomical landmark as a way to quantitatively assess accuracy of the representation (Figure 2). In addition, an easily identifiable bone fragment within the fracture was measured for each case as a pathological measurement. First, digital measurements were taken using Change PACS. The measurements were then repeated on the 3D renderings after segmentation using the respective segmentation software. Then, separately, physical measurements taken from the 3D printed models were recorded using high-grade digital calipers (PEC Tools, Santa Monica, CA, USA) by a second observer who was blinded to the model type (15). Measurements were rounded to the nearest tenth of a millimeter on both the digital measurements and the physical measurements. The difference between the measurement recorded on the CT scan and the physical 3D printed model was recorded and used for data analysis as the measurement error; absolute values were also recorded to analyze errors without direction bias.
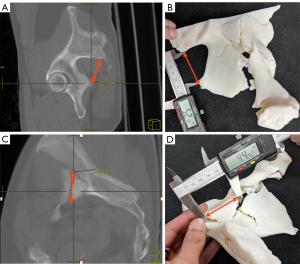
Clinical evaluation
The models were evaluated using a 15-question survey (10 point Likert scale, with 10 being a positive response, and 1 being a negative response) adapted from previous observations of anatomical modeling within surgical planning (11,16). Five physicians (one orthopedic surgeon involved in the operation, three radiologists, and an anesthesiologist) provided clinical feedback about the models. Questions include topics such as accuracy, production time, assistance in planning, efficiency, inventory management, potential complication avoidance, recommendation for future operations (Appendix 1).
Statistical analysis
The material costs associated with manufacturing were calculated based on the amount of material used and cost per weight of the material. Two one-way ANOVAs were used to analyze the mean absolute error values for the distance recorded between the PIIS and the ischial spine as well as the error values of the fracture fragment for each of the 4 printers used with a significance level of P≤0.05. A one-way ANOVA was used to compare the survey results of the different models produced, with a significance level of P≤0.05.
In addition, the data was also analyzed using a Bland-Altman analysis (17,18). Pearson product-moment correlation coefficient was calculated to examine the correlations between the difference and the mean of the difference from the mean values and explain the agreement between the two quantitative measures taken. A P value ≤0.05 was considered statistically significant for the measurement of proportional bias.
Results
Model descriptions
The following measurements were recorded when measuring the distance from the PIIS to the ischial spine directly on the CT images: Model 1 at 47.0 mm, Model 2 at 53.7 mm, Model 3 at 51.5 mm, Model 4 at 52.5 mm, and Model 5 at 70.0 mm.
The fracture fragments used to measure the precision of the printing method varied depending on the nature of the fracture and was measured directly on the CT imaging data. Model 1 was a both-column acetabular fracture, and the measured fragment was posterior and superior to the acetabulum and measured 63.2 mm. Model 2 was an acetabular fracture with a protrusion, with the fracture fragment located anterior to the acetabulum on the superior aspect measuring 35.4 mm. Model 3 was a gunshot acetabular fracture with the measured fragment posterior to the acetabulum and measuring 41.4 mm. Model 4 was a both column acetabular fracture with the measured fragment being a triangular fragment along the inner iliac wing and measured 44.4 mm. Model 5 was a posterior acetabular fracture with the fragment being superior to the hip joint and measured 38.1 mm.
Measurements of the distance between the PIIS to the ischial spine and the acetabular fracture fragment were assessed with the respective software that was used for the segmentation process and can be seen in Table 2. The mean absolute error from the software measurements was found not to be statistically significant between software types for both the PIIS to ischial spine distance and the fracture fragment distance, F(3,19) =0.426, P=0.737.
Table 2
Model | Original CT (mm) | 3D Slicer Segmentation (mm) | Mimics Segmentation (mm) | |||||
---|---|---|---|---|---|---|---|---|
PIIS to IS | Fracture | PIIS to IS | Fracture | PIIS to IS | Fracture | |||
1 | 47.0 | 63.2 | 47.6 | 62.9 | 47.3 | 62.4 | ||
2 | 53.7 | 35.4 | 53.4 | 34.6 | 53.6 | 34.8 | ||
3 | 51.5 | 41.4 | 52.0 | 40.7 | 52.2 | 41.4 | ||
4 | 52.5 | 44.4 | 52.2 | 44.1 | 52.8 | 44.9 | ||
5 | 70.0 | 38.1 | 69.1 | 37.7 | 69.6 | 38.0 |
Measurement comparison
The one-way ANOVA showed that there was no significant difference in the measurement error between the models produced from either method, F(3,19) =1.459, P=0.263 (Figure 3A). When comparing the error in the fracture fragment measurement between the models, there was a significant difference between the Objet and the other three methods, F(3,19) =5.768, P=0.007. The Objet models had significantly less error than the uPrint models (P=0.026), Form 2 models (P=0.026), and the Ultimaker models (P=0.010) (Figure 3B). Examination of the distributions of measurement errors indicate physical models both over- and under-estimated the true distance with a slight bias towards over-estimation on all four printers (Figure 4).
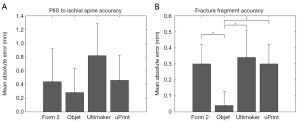
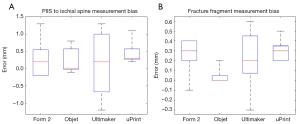
The results of the Bland-Altman analysis show that the differences between production methods resulted in similar results when comparing the representation of the general anatomy as well as the fracture fragment. In each Bland-Altman plot produced, a majority of the values fell within two standard deviations of the mean. For all the Bland-Altman analyses, there were no significant linear regression values for the PIIS to ischial spine measurement or the fracture measurement (r=0.022 and r=0.103, respectively) or indications of proportional bias (P=0.927 and P=0.625, respectively) (Figure 5).
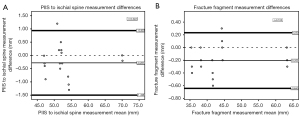
Clinical survey comparison
For the survey analysis, only questions that were relevant to the study and received more than one response were included in the analysis (Table 3). This meant the exclusion of Question 5, Question 8, Question 9, Question 10, Question 11, and Question 12. These questions were outside the scope of the study and/or did not receive sufficient feedback (N≤1).
Table 3
Model | (Q1) Anatomical accuracy, N=4 | (Q2) Clinical significance of anatomy, N=4 | (Q3) Pathological accuracy, N=4 | (Q4) Clinical significance of fracture, N=4 | (Q6) Improvement from CT alone, N=4 | (Q7) Utility for pre-operative planning, N=2 | (Q13) Recommend for future use, N=2 | (Q14) Help communicate with patients, N=3 | (Q15) Overall satisfaction, N=5 |
---|---|---|---|---|---|---|---|---|---|
Form 2 | 8.55±0.94 | 8.80±1.44 | 7.50±1.32 | 7.20±2.21 | 6.72±1.41 | 8.00±0.00 | 10.0±0.00 | 9.00±1.46 | 8.42±1.50 |
Objet | 8.65±0.93 | 8.95±1.23 | 8.30±0.8** | 8.00±1.97 | 6.61±1.29 | 8.00±0.00 | 10.0±0.00 | 9.00±1.46 | 9.00±0.78 |
Ultimaker | 8.45±0.94 | 8.70±1.49 | 7.35±1.23*,** | 7.20±2.21 | 6.72±1.41 | 8.00±0.00 | 10.0±0.00 | 9.00±1.46 | 8.33±1.49 |
uPrint | 8.65±0.93 | 8.95±1.23 | 8.25±0.85* | 8.00±1.97 | 6.61±1.29 | 8.00±0.00 | 10.0±0.00 | 9.00±1.46 | 9.00±0.78 |
All questions are based on a 1–10 Likert scale where 1 indicates not very helpful/Useful and 10 indicates very helpful/useful. Significant differences between the results are denoted with by *(between the uPrint and the Ultimaker) and **(between the Objet and the Ultimaker).
The one-way ANOVA of the survey data indicated that there was a significant difference between model types F(3,79)=4.256, P=0.008. Tukey post-hoc analysis showed that there was a significant difference in the perceived representation of the fracture between the uPrint (high-end FDM) and the Ultimaker (low-cost FDM) (P=0.047), as well as the Objet (high-end resin) and Ultimaker (low-cost FDM) (P=0.032). There were no significant differences found between the other questions and model types included in the survey. The low sample size of the survey and number of responses indicate the survey is subject to potential bias and will be addressed more thoroughly in the discussion.
Discussion
The results of this study found that the type of printer used to develop acetabular fracture models has a slight effect on the final product. When analyzing the mean absolute error of the distance between the PIIS and the ischial spine compared to the original CT, there were no significant differences between any of the models produced (P=0.263). However, the high-end resin (Objet) printer had a significantly different mean absolute error of the fracture fragment measurement than the other printers (P=0.007) indicating higher accuracy, which was expected. Of the 9 different survey questions used for analysis, there was only a significantly lower difference in the representation of the pathology when using the low-cost FDM method (7.35±1.23) when compared to the two different high-end prints (FDM =8.25±0.85, Resin =8.30±0.80).
The purpose of this study was to observe if acetabular fracture models could be produced using more affordable methods while still maintaining the same clinical validity as high-end models. Comparisons made between the pathological measurements indicated that the models produced by the high-end resin (Objet) printer were significantly more precise than the models produced by all 3 other printers (P=0.007). In this case, the error was 0.3 mm, which was only 0.48% of the largest fracture length (63.2 mm) and 0.85% of the smallest fracture length (35.4 mm), and thus unlikely to be clinically significant. While this small error may be negligible in structures as large as the acetabulum, it could prove significant when modeling small structures or vasculature in pediatric patients. Furthermore, this small difference in the fracture pattern was also reflected by the physicians who provided feedback on the anatomic models. There was a significant difference in Question 3, which asked “Is the model an accurate representation of the pathology?” (P=0.047). However, the subsequent question in the survey asked “If there are inaccuracies to the pathology representation, are they clinically significant to you?” and this was found not to be significant (P=0.412). This suggests that the model differences produced by each method (both high-end and low-cost) are not significant enough to result in a different clinical outcome, or that they still convey the same amount of information about the fracture and can still be utilized for things such as surgical planning. Currently, 3D Slicer is intended for research purposes, rather than clinical application like Mimics is. However, the results of this study indicate that the open-source software could offer some utility to institutions that do not have the resources for proprietary software.
The results of the survey suggest that anatomical models can play a valuable role in pre-surgical planning regardless of the methodology used for development. All of the acetabular models were found to be accurate representations of the general anatomy (average of 8.58±0.94), and that any inaccuracies between the imaging data and the model itself were not clinically relevant for both the general anatomy (8.85±1.35) and for the fracture itself (7.6±2.09). Additionally, the 3D model provides a new and useful perspective compared to 2D CT images alone (6.67±1.35) and that there was an overall satisfaction with the final product (8.69±1.14). However, one major limitation from this study is the quality of information that can be drawn from the survey portion. The models were reviewed by 5 participants in different fields of medicine, not all of which would use the model for the intended surgical planning purpose and may be subject to a fair amount of bias. The survey suggests that the models are representative and accurate, however may not be conclusive from this study alone.
Cost has often been cited as a barrier to entry for 3D printing, which is especially true for developing countries (19). Any usage after the initial purchase is reduced to only the cost of the material, which for this experiment ranged from $3.30 to $4.70 for the low-cost FDM models. Many of the questions included on the survey indicate that the models produced are both accurate representations of the overall structure (average score of 8.58) and a useful step in planning the operation (average score of 8). This indicates that for as low as $3.30, it is possible to create an acetabular model that could both improve surgery preparation as well as teach students about complex cases that may arise. In one educational application, a model developed for ~$10 was estimated to have saved 45 minutes within the operating room, and was equivalent to saving $2,700 for the hospital (8). While these numbers are estimates, they help to show how the monetary benefits from this type of education would be difficult to calculate but likely outweigh the cost of the material for facilities with less access to resources.
While some hospitals are well funded and can afford to use equipment regardless of its cost for medical 3D printing, hospitals with less abundant resources likely do not have this option. This study was designed to test the entire production method from segmentation to final product with this application in mind. A portion of error was included as a result of both segmentation methods, however the difference between them was not significant. This suggests that the main source of difference between the final product is the printer used for manufacturing. Based on the hardware limits of the 3D printers, it has been reported that models produced using SLA methods/resin based material would likely have the most accurate results in reflecting the anatomy (20). However, the error differences found in this study were extremely small, often times less than 1mm, and would likely not play a significant role in surgical planning for orthopedic purposes. Even with the slight differences in accuracy, the low-cost FDM model had similar physician feedback like what has been reported in other studies, and should encourage its usage in more countries (21,22).
Focusing specifically on the acetabulum for model development allows for easier comparison between production types, however, makes it more difficult to apply the results to models of different structures such as the heart or the liver for complex tumor representations. 3D printing specifically for orthopedics will likely have similar results, but more complex multi-part models may need access to more material textures and colors. This type of material mixing currently does not easily exist for low-cost 3D printing. High-end printers like the Objet that are capable of mixing materials have much more versatility in what types of models can be produced. The results of this study only reflect single material anatomical models but could be replicated with a low-cost multi-material printer once it becomes more accessible.
As previous research has indicated, the utility of 3D printed models is only beginning to be explored due to the number of factors that can influence model utilization. This includes the purpose of the model, imaging techniques used to drive segmentation, segmentation expertise and software used, and printer specifications (9). In some cases, information such as operating room time and follow-up time were recorded to observe the impact of the models (8,23,24). However, a majority of the articles involving anatomical modeling report little to no qualitative information (25-28). One reason for the large variety of measured outcomes is that different operations will have different measures of success. The differences in model purpose make comparing the effectiveness of each model unique to its application, and the results from one study may not be generalizable to other studies.
These findings indicate that low-cost methods of developing 3D printed acetabular fracture and possibly other models may be sufficient to produce accurate representations that provide substantial clinical value to the surgeons. Therefore, the practice of printing models to improve surgical outcomes should be feasible for clinics with limited funding. Future studies should determine the extent to which the low-cost application is feasible through implementation in different regions of the body as well as impacts on different operations outcomes and which ones are relevant to their study.
Conclusions
This study observed 3D printed acetabular fracture models made using different production methods, their representations of the fracture, and potential utility as a method of surgical planning. The results found that while there was a minor difference in the accuracy between the methods of production, there was no difference between the quality of information that the models provided. Hospitals with less access to resources could potentially use this information to consider including low-cost 3D printed anatomic models for their complex surgical procedures.
Acknowledgments
We would like to thank Dr. Ka-Chun “Joseph” Siu (University of Nebraska Medical Center) for his feedback on the study.
Funding: This research was partially funded by the National Institute of Neurological Disorders and Stroke of the National Institutes of Health under Award Number R01NS114282 and the Biomechanical Rehabilitation and Manufacturing Initiative (BRMI), as well as the NASA Nebraska Space Grant.
Footnote
Reporting Checklist: The authors have completed the MDAR reporting checklist. Available at https://atm.amegroups.com/article/view/10.21037/atm-21-5069/rc
Data Sharing Statement: Available at https://atm.amegroups.com/article/view/10.21037/atm-21-5069/dss
Peer Review File: Available at https://atm.amegroups.com/article/view/10.21037/atm-21-5069/prf
Conflicts of Interest: All authors have completed the ICMJE uniform disclosure form (available at https://atm.amegroups.com/article/view/10.21037/atm-21-5069/coif). The authors have no conflicts of interest to declare.
Ethical Statement: The authors are accountable for all aspects of the work in ensuring that questions related to the accuracy or integrity of any part of the work are appropriately investigated and resolved. The study was conducted in accordance with the Declaration of Helsinki (as revised in 2013). This study was approved through the IRB at the University of Nebraska Medical Center (258-18-EP) with waiver of consent due to the data being deidentified and accessed retrospectively
Open Access Statement: This is an Open Access article distributed in accordance with the Creative Commons Attribution-NonCommercial-NoDerivs 4.0 International License (CC BY-NC-ND 4.0), which permits the non-commercial replication and distribution of the article with the strict proviso that no changes or edits are made and the original work is properly cited (including links to both the formal publication through the relevant DOI and the license). See: https://creativecommons.org/licenses/by-nc-nd/4.0/.
References
- Rinne PP, Laitinen MK, Kannus P, et al. The incidence of pelvic fractures and related surgery in the Finnish adult population: a nationwide study of 33,469 patients between 1997 and 2014. Acta Orthop 2020;91:587-92. [Crossref] [PubMed]
- Mauffrey C, Hao J, Cuellar DO 3rd, et al. The epidemiology and injury patterns of acetabular fractures: are the USA and China comparable? Clin Orthop Relat Res 2014;472:3332-7. [Crossref] [PubMed]
- Boudissa M, Francony F, Kerschbaumer G, et al. Epidemiology and treatment of acetabular fractures in a level-1 trauma centre: Retrospective study of 414 patients over 10 years. Orthop Traumatol Surg Res 2017;103:335-9. [Crossref] [PubMed]
- Whiting PS, Anderson DR, Galat DD, et al. State of Pelvic and Acetabular Surgery in the Developing World: A Global Survey of Orthopaedic Surgeons at Surgical Implant Generation Network (SIGN) Hospitals. J Orthop Trauma 2017;31:e217-23. [Crossref] [PubMed]
- Mesbahi SAR, Ghaemmaghami A, Ghaemmaghami S, et al. Outcome after Surgical Management of Acetabular Fractures: A 7-Year Experience. Bull Emerg Trauma 2018;6:37-44. [Crossref] [PubMed]
- Jacobs S, Grunert R, Mohr FW, et al. 3D-Imaging of cardiac structures using 3D heart models for planning in heart surgery: a preliminary study. Interact Cardiovasc Thorac Surg 2008;7:6-9. [Crossref] [PubMed]
- Salazar D, Huff TJ, Cramer J, et al. Use of a three-dimensional printed anatomical model for tumor management in a pediatric patient. SAGE Open Med Case Rep 2020;8:2050313X20927600.
- Cherkasskiy L, Caffrey JP, Szewczyk AF, et al. Patient-specific 3D models aid planning for triplane proximal femoral osteotomy in slipped capital femoral epiphysis. J Child Orthop 2017;11:147-53. [Crossref] [PubMed]
- Mitsouras D, Liacouras P, Imanzadeh A, et al. Medical 3D Printing for the Radiologist. Radiographics 2015;35:1965-88. [Crossref] [PubMed]
- Zheng YX, Yu DF, Zhao JG, et al. 3D Printout Models vs. 3D-Rendered Images: Which Is Better for Preoperative Planning? J Surg Educ 2016;73:518-23. [Crossref] [PubMed]
- Birbara NS, Otton JM, Pather N. 3D Modelling and Printing Technology to Produce Patient-Specific 3D Models. Heart Lung Circ 2019;28:302-13. [Crossref] [PubMed]
- Martin CM, Roach VA, Nguyen N, et al. Comparison of 3D reconstructive technologies used for morphometric research and the translation of knowledge using a decision matrix. Anat Sci Educ 2013;6:393-403. [Crossref] [PubMed]
- Ripley B, Levin D, Kelil T, et al. 3D printing from MRI Data: Harnessing strengths and minimizing weaknesses. J Magn Reson Imaging 2017;45:635-45. [Crossref] [PubMed]
- Fedorov A, Beichel R, Kalpathy-Cramer J, et al. 3D Slicer as an image computing platform for the Quantitative Imaging Network. Magn Reson Imaging 2012;30:1323-41. [Crossref] [PubMed]
- Leng S, McGee K, Morris J, et al. Anatomic modeling using 3D printing: quality assurance and optimization. 3D Print Med 2017;3:6.
- Bagaria V, Chaudhary K. A paradigm shift in surgical planning and simulation using 3Dgraphy: Experience of first 50 surgeries done using 3D-printed biomodels. Injury 2017;48:2501-8. [Crossref] [PubMed]
- Zuniga J, Katsavelis D, Peck J, et al. Cyborg beast: a low-cost 3d-printed prosthetic hand for children with upper-limb differences. BMC Res Notes 2015;8:10. [Crossref] [PubMed]
- Bland JM, Altman DG. Statistical methods for assessing agreement between two methods of clinical measurement. Lancet 1986;1:307-10. [Crossref] [PubMed]
- Tetsworth KD, Mettyas T. Overview of Emerging Technology in Orthopedic Surgery. Tech Orthop 2016;31:143-52. [Crossref]
- AlAli AB, Griffin MF, Butler PE. Three-Dimensional Printing Surgical Applications. Eplasty 2015;15:e37. [PubMed]
- Mottl-Link S, Hübler M, Kühne T, et al. Physical models aiding in complex congenital heart surgery. Ann Thorac Surg 2008;86:273-7. [Crossref] [PubMed]
- Velasco I, Vahdani S, Ramos H. Low-cost Method for Obtaining Medical Rapid Prototyping Using Desktop 3D printing: A Novel Technique for Mandibular Reconstruction Planning. J Clin Exp Dent 2017;9:e1103-8. [Crossref] [PubMed]
- Zheng W, Tao Z, Lou Y, et al. Comparison of the Conventional Surgery and the Surgery Assisted by 3d Printing Technology in the Treatment of Calcaneal Fractures. J Invest Surg 2018;31:557-67. [Crossref] [PubMed]
- Martelli N, Serrano C, van den Brink H, et al. Advantages and disadvantages of 3-dimensional printing in surgery: A systematic review. Surgery 2016;159:1485-500. [Crossref] [PubMed]
- Anderson JR, Thompson WL, Alkattan AK, et al. Three-dimensional printing of anatomically accurate, patient specific intracranial aneurysm models. J Neurointerv Surg 2016;8:517-20. [Crossref] [PubMed]
- Xie M, Tang K, Yuan C. 3D printing lunate prosthesis for stage IIIc Kienböck’s disease: a case report. Arch Orthop Trauma Surg 2018;138:447-51. [Crossref] [PubMed]
- Igami T, Nakamura Y, Hirose T, et al. Application of a three-dimensional print of a liver in hepatectomy for small tumors invisible by intraoperative ultrasonography: preliminary experience. World J Surg 2014;38:3163-6. [Crossref] [PubMed]
- Schmauss D, Haeberle S, Hagl C, et al. Three-dimensional printing in cardiac surgery and interventional cardiology: a single-centre experience. Eur J Cardiothorac Surg 2015;47:1044-52. [Crossref] [PubMed]