Electroacupuncture attenuates spared nerve injury-induced neuropathic pain possibly by promoting the progression of AMPK/mTOR-mediated autophagy in spinal microglia
Highlight box
Key findings
• Our work found the possible mechanism of the analgesic impact of EA in neuropathic pain is partly through AMPK/mTOR pathway and autophagy induction of microglia in spinal dorsal horn.
What is known and what is new?
• Electroacupuncture is an effective way to treat neuropathic pain. Neuropathic pain is associated with impaired autophagy and induction of autophagy would lead to analgesic effect.
• The analgesic effect of electroacupuncture in neuropathic pain is partly through activation of AMPK/mTOR pathway and autophagy induction of microglia in spinal dorsal horn.
What is the implication, and what should change now?
• Our research provides a basis and guidance for autophagy-targeting pathway and EA application in pain therapy, offering new targets and ideas for pain therapy.
Introduction
Neuropathic pain (NP) syndrome is caused by central or peripheral nerve injury and primarily manifests as hyperalgesia, spontaneous pain, and allodynia. Its pathology involves the activation of glial cells, such as microglial cells and astrocytes, in the spinal cord, which enter a hypertrophic phase during both the NP induction and maintenance periods (1,2). Treatment of NP is still a challenge because many patients do not experience sufficient pain relief, as determined from clinical experience and from clinical trial outcomes. Various types of drugs, including antidepressants with norepinephrine and serotonin reuptake inhibition, calcium channel α2-δ ligands, opioid analgesics, and topical lidocaine, have been shown to have consistent efficacy in clinical patients (3,4). However, pharmacological treatments have various side effects, which often causes poor compliance of patients. Electroacupuncture (EA) is a common form of acupuncture involving an electric current under precisely controlled parameters (5), which makes this method more highly reproducible and superior to manual acupuncture (6). It is widely used in China and all over the world because it is safer, more effective, and has fewer side effects than other treatments. Although EA is an effective way of treating all types of pain, its exact mechanism of action in NP requires further investigation (7).
Autophagy is a crucial physiological process that is necessary for cell differentiation, homeostasis, as well as survival, and is induced by factors including environmental or cellular stresses (8,9). Autophagic dysfunction is associated with neurodegenerative diseases such as Huntington’s disease, spinocerebellar ataxia, and Parkinson’s disease, which are associated with an accumulation of toxic protein due to ineffective lysosomal clearance by autophagy (10). A previous study has reported altered expression of autophagy-associated proteins in rat models of NP, indicating an impaired autophagy flux (11). Other reports have mentioned that intrathecal injection of an autophagy blocker in mice induced significant mechanical hypersensitivity, and treatment with the autophagy inducer rapamycin could ameliorate NP by activating autophagy in the spinal cord, suggesting that NP may have occurred due to impaired autophagy (12,13).
Spinal microglial activation has been reported to play a critical role in NP occurrence, particularly during the initial stages (14-16). EA has been reported to alleviate both tactile allodynia and thermal hyperalgesia by inhibiting the activation of spinal microglia (17,18). Furthermore, studies have also shown that peripheral nerve injury could induce changes in the activation of autophagy in microglia (11,19). Autophagy levels can be modulated mainly through the AMPK/mTOR pathway, where AMPK activation can alleviate chronic pain via inhibition of the mTOR signaling pathway (20). Moreover, the expression of AMPK in the hypothalamus is related to the analgesic effect of EA (21).
In the present study, we hypothesized that EA might attenuate NP by promoting AMPK/mTOR-mediated autophagy in microglia. To confirm this hypothesis, we first tested the analgesic effects of EA in NP rats. Next, we successfully demonstrated that peripheral nerve injury could induce microglial cell activation and increases the level of autophagy. Furthermore, we explored the causal relationship between EA-induced inhibition of NP and increased autophagic levels by using the AMPK inhibitor. We present the following article in accordance with the ARRIVE reporting checklist (available at https://atm.amegroups.com/article/view/10.21037/atm-22-5273/rc).
Methods
Animal and surgery
The animal experiments were carried out using adult male Sprague-Dawley rats (weight 160–180 g, Nanjing University of Chinese Medicine). A protocol was prepared before the study without registration. Experiments were performed under a project license (No. ACU210502) granted by the Committee on animal experimentation at Nanjing University of Chinese Medicine, in compliance with national guidelines for the care and use of animals. For at least 3 days before beginning the experiment, the rats were housed with free access to food and water in light-controlled rooms (12/12 h light/dark cycle) maintained at a temperature of 23±2 ℃. The method as described by Cichon et al. was utilized to induce NP by spared nerve injury (SNI) (22). Briefly, anesthetization of the rats was first achieved using isoflurane (2–3%), followed by exposing the left lateral sciatic nerve and its three terminal branches (the sural, common peroneal, and tibial nerves). The left lateral common peroneal and tibial nerves were tightly ligated with silk sutures and sectioned at the distal to the ligation point, with at least 2–4 mm of the distal nerve stump removed while keeping the sural nerve left intact. Then, under sterile conditions, the muscle and skin tissues were closed. We used similar surgical methods to process the sham surgery rats, except for leaving both the tibial and common peroneal nerves intact.
Analysis of EA stimulation
To perform the EA therapy, the rats were restrained in homemade opaque cloth bags with their hind legs exposed. Then, two acupuncture needles (made of stainless steel) were inserted at least 6–7 mm at two acupoints ipsilateral to the injured side. We inserted one needle into ST36, 5 mm lateral to an anterior tubercle of the tibia labeled by a notch. Another needle was placed at the Huantiao acupoint (GB30) (i.e., on the intersection of the outermost one-third and the middle one-third of the line), which connects the most prominent point of the femur and the sacral hiatus. These two acupoints were selected, as stimulation at the “Zusanli” (ST36) and “Huantiao” (GB30) acupoints have already been reported to induce analgesia in various pain models (23-25). Han’s Acupoint Nerve Stimulator (HANS, 200A, Nanjing Jisheng Medical Technology Co., Ltd., China) was used to generate the stimulation square waves of EA. One week after SNI, the rats were stimulated with EA for 30 minutes once daily for 7 consecutive days at an EA stimulation frequency of 2 Hz, while increasing the stimulation intensity stepwise from 1 to 2 to 3 mA every 10 min. An acupuncture needle was only shallowly inserted in ST36 and GB30 without electrical stimulation for the SNI + acupuncture (SNI + A) group.
Intrathecal drug administration
As described previously (26), intrathecal administration was performed under isoflurane (2.5%) anesthesia by lumbar puncture, which involved the insertion of a 26G gauge needle between the L5 and L6 vertebrae. An AMPK inhibitor compound C dose of approximately 10 µL (0.2 µmol/kg, HY13418A, MedChemExpress company, USA) diluted in dimethylsulfoxide (DMSO) was administered 1 h before EA stimulation once daily for 7 days. DMSO alone was used for the control injections. An injection-induced tail flick was observed to ensure the quality of each injection.
Paw withdrawal threshold (PWT) measurement
The hypersensitivity towards punctuates mechanical stimulation with von-Frey filaments (0.38, 0.57, 1.23, 1.83, 3.66, 5.93, 9.13, and 13.1 g) has been determined previously by adopting the up-down method (27). We considered an abrupt withdrawal of paws, licking, and shaking as positive responses. Dixon’s approach and formula were applied to measure the PWT. To minimization of experimenter bias, the investigator carrying out the behavioral tasks was blinded to the treatment conditions.
Immunofluorescence analysis
Using pentobarbital, the animals were anesthetized and perfused with 20 mL of 0.1 M phosphate-buffered saline (PBS) and 25 mL of fixative-containing saturated picric acid (14%, v/v) and formaldehyde (4%) in PBS at 4 ℃. We embedded lumbar spinal cord tissues, cut them into sections (thickness: 15 µm), and placed them onto slides. The slides were then blocked with 0.2% Triton X-100 and 5% normal donkey serum dissolved in PBS for 1 hour at room temperature, before being incubated with primary antibodies overnight at 4 ℃. A solution containing sodium azide (0.01%), bovine serum albumin (1%), and Triton X-100 (0.3%) in PBS was used to dilute the primary antibodies to their final working concentrations. FluoroShield histology mounting medium (Sigma-Aldrich, USA) was applied to the slides following 45 minutes of incubation with secondary antibodies. The primary antibodies used in this study were as follows: goat anti-GFAP (1:50, Abcam, ab53554), mouse anti-NeuN (1:20, Abcam, ab104224), mouse anti-CD11b (1:20, Abcam, ab1211), rabbit anti-p62 (1:50, Abcam, ab91526), and rabbit anti-goat (Alexa Fluor® 488) (1:200, Abcam, ab150192). Subsequently, the following secondary antibodies were utilized in this investigation: rhodamine Red-X-conjugated AffiniPure donkey anti-rabbit IgG (H + L) (1:200, Jackson ImmunoResearch, West Grove, PA, 711-295-152) and fluorescein-conjugated AffiniPure donkey anti-mouse IgG (H + L) (1:200, Jackson ImmunoResearch, 711-095-151). We captured images using a fluorescence microscope (Olympus BX51 microscope system, Melville, NY). The integrated optical density (IOD), a parameter in immunostaining, was analyzed using Image J software (NIH, Bethesda, MD). Areas with overlapping similarities were evaluated between distinct animals. We also subtracted any background signals arising from each subsequent slice. The average IOD of the sham-operated rats was also determined. We then normalized the IOD ratio among the SNI rats to the average values of the sham-operated rats to illustrate the fold value of the sham rats to conduct a comparison. Here, data analysis was performed with the investigator blinded to the experimental groups.
Western blotting
For western blot assays, ipsilateral segments of the lumbar spine (including L4–L5) from the rats were isolated, followed by homogenization in an ice-cold radio immunoprecipitation assay (RIPA) buffer. Using a detergent-compatible protein assay and bovine serum albumin standard, the protein concentration was determined after centrifugation of the homogenates. Protein separations were performed on 10% or 12% sodium dodecyl sulfate-polyacrylamide gel electrophoresis and transferred to nitrocellulose membranes. Incubation of the membranes with the primary antibody was carried out overnight at 4 ℃ after blocking with skim milk for 1 hour. Incubation with the secondary antibody was performed at room temperature for 2 hours after washing the membranes three times with Tris buffered saline Tween (TBST). An enhanced chemiluminescence kit (Amersham, USA) was used to detect the immunoreactivity of membranes after washing with TBST three times.
The antibodies used in this study were against p62 (1:1,000, Abcam, UK, ab91526), Beclin-1 (1:1,000, Abcam, UK, ab210498), LC3 (1:2,000, Abcam, UK, ab192890), AMPK (1:1,000, Cell Signaling Technology, USA, 5831S), p-AMPK (1:1,000, Cell Signaling Technology, USA, 2535S), and mTOR (1:1,000, Cell Signaling Technology, USA, 5174). We then quantified the intensity of immunoreactive bands of interest on autoradiograms using Image J software. Glyceraldehyde-3-phosphate dehydrogenase (GAPDH) was applied as a control for the loading of protein samples.
Transmission electron microscopy (TEM)
For TEM analysis, the ipsilateral lumbar spine segments (including L4 and L5 segments) of the rats were harvested, cut into 1 mm3 tissue samples, fixed in glutaraldehyde (2.5%) for 2 h at room temperature, and rinsed three times with PBS (10 min each time). After fixation for 1.5 h in Osmium acid solution (1%) at 4 ℃, the fixative solution was removed, and the samples were washed three times in PBS (15 min each time). The samples were then dehydrated using a series of gradient alcohol, embedded, sectioned, and then observed and photographed using an electron microscope [Mintz Precision Instruments (Shanghai) Co., Ltd., China]. We also detected autophagosomes using a transmission electron microscope [Mintz Precision Instruments (Shanghai) Co., Ltd.].
Statistical analysis
In the figure legends, we described the statistical methods used for each assay. The data shown are representative results of experiments involving a minimum of three biological replicates of comparable outcomes. Statistical analyses were carried out using GraphPad Prism 5.0 software. Bonferroni correction was utilized for multiple comparisons in analysis of variance (ANOVA). We also conducted two-tailed tests and the data was presented as the mean ± standard error of the mean (SEM), and P<0.05 was considered the criterion of statistical significance in all tests.
Results
Mechanical hypersensitivity was attenuated in SNI rats through EA stimulation
Our primary goal was to investigate whether EA attenuates neuropathic mechanical hypersensitivity among SNI rats. To achieve this, the SNI rats were administered EA or acupuncture from day 7 until day 14 post-SNI. The sham-operated rats, who did not receive any treatment, were considered surgical controls. It was observed that during testing periods, the PWT in the sham-operated rats did not change considerably from the baseline value. Furthermore, it was also observed that SNI induced severe mechanical allodynia on day 7 after surgery, which persisted until day 14 (P<0.05, two-way mixed-model ANOVA). However, EA significantly increased the PWT on day 14 after surgery (P<0.05, two-way mixed-model ANOVA). The PWT in SNI rats was not significantly increased by acupuncture (Figure 1A).
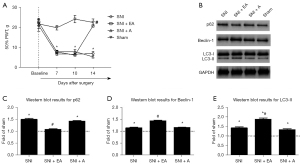
Analyzing the expression of autophagy-related proteins from the spinal dorsal horn upon EA stimulation
On day 14 following SNI, spinal dorsal horn cells ipsilateral to the injured side were collected to evaluate the effect of EA on the expression of autophagy-related proteins (i.e., p62, Beclin-1, and LC3-II). The ubiquitin-binding protein p62 is incorporated into autophagosomes by directly binding to LC3 and is efficiently degraded in autolysosomes (28,29). Therefore, autophagy is impaired with p62 accumulation. The Beclin-1 protein mediates the initiation step and is crucial for autophagosome formation (30). LC3-I, as the unconjugated cytosolic form, transforms to LC3-II after being recruited to the autophagosomal membrane upon conjugation to phosphatidylethanolamine. LC3-II demonstrates greater electrophoretic mobility. The ratio of LC3-II to LC3-I is thought to be a reliable indicator of autophagy (30).
Figure 1B shows the illustrative immunoblots of p62, Beclin-1, and LC3-II expression in the ipsilateral lumbar spinal cord. We observed that the levels of p62 were considerably elevated on day 14 after SNI compared to the sham group (P<0.05, one-way ANOVA; Figure 1C). Treatment with EA attenuated the increase in p62 expression, and the level of p62 in the acupuncture group did not differ significantly from that in the SNI group (Figure 1C). A significant increase in Beclin-1 and LC3-II expression levels was observed on day 14 in the SNI group (P<0.05, one-way ANOVA). However, the expression levels of Beclin-1 and LC3-II in the SNI + EA group were considerably elevated compared to the SNI group (P<0.05, one-way ANOVA; Figure 1D,1E). As a result of the enhanced expression of p62, autophagy was blocked after SNI. The elevated levels of Beclin-1 and LC3-II might be caused by impaired autophagosome clearance instead of autophagy induction.
SNI induces microglial activation in the spinal dorsal horn accompanied by impaired autophagy
We carried out immunofluorescence staining using the antibodies against p62 and the astrocytes marker GFAP, the microglia marker CD11b, and the neuronal marker NeuN. As shown in Figure 2A, SNI induced the activation of both astrocytes and microglia in the spinal dorsal horn, signified by hyperplasia and hypertrophy with enhanced GFAP or CD11b expression compared to the sham surgery rats. Compared to the sham-operated rats, p62 immunoreactivity was increased in the dorsal horn of the spinal cords among the SNI rats, indicating impaired autophagy among the SNI rats. The expression of NeuN did not change significantly after SNI.
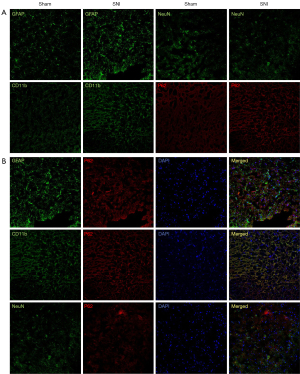
The cellular localization of p62 expression was studied by the double immunofluorescence labeling of p62 with intracellular biomarkers of neurons, astrocytes, and microglia in the ipsilateral dorsal horn on day 14 day after SNI. P62 (red) was colocalized with CD11b (green) but not with GFAP (green) or NeuN (green) (Figure 2B). Thus, the double-labeling experiment demonstrated that p62 was expressed in most microglia.
EA in SNI rats induced autophagosome formation in the microglia of the spinal dorsal horn (detected by TEM)
The spinal dorsal horn samples from the four groups (SNI, SNI + EA, SNI + A, and sham) were collected on day 14 of post-surgery after quantifying the PWT. TEM of the autophagosome in the microglia of the spinal dorsal horn showed that autophagosome formation was significantly enhanced in SNI rats compared to the sham-operated rats, whereas EA in SNI rats markedly increased autophagosome formation compared to the SNI group (Figure 3). SNI rats were not significantly affected by acupuncture in terms of autophagosome formation, and might have exhibited increased autophagosomes due to impaired clearance rather than autophagy induction. These results indicate that EA could induce autophagy progression in the microglia of the spinal dorsal horn in SNI rats.
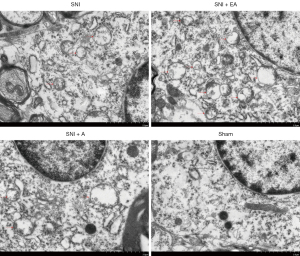
EA could attenuate NP by promoting AMPK/mTOR-mediated autophagy of microglia in the spinal cord
Compound C, an AMPK inhibitor, was injected intrathecally to determine the causal relationship between EA-induced inhibition of NP and enhanced autophagy in the microglia, and DMSO, a solvent for compound C, was used as a control. From day 5 after surgery, the PWT decreased with SNI, and the lowest point was reached on day 7 following surgery and remained at a low level until day 14 after surgery (P<0.05, two-way mixed-model ANOVA; Figure 4). After surgery, EA administered between days 7 and 14 substantially increased the PWT on day 14 after surgery, which was reversed by the intrathecal injection of compound C 1 h before every EA treatment (P<0.05, two-way mixed-model ANOVA; Figure 4). As a control, DMSO did not notably influence the analgesic effect of EA in the SNI rats.
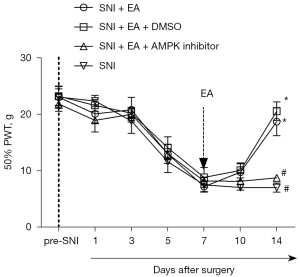
Researchers also examined the role of AMPK/mTOR signaling in EA-induced autophagy by measuring autophagy-related proteins, including p62, Beclin-1, LC3-II, and p-AMPK/AMPK, as well as p-mTOR/mTOR expression in the ipsilateral lumbar spinal cord of SNI rats with or without EA or compound C treatment on day 14 post-surgery (Figure 5). Compared with the SNI group, EA considerably reduced the expressions of p62 and p-mTOR/mTOR, leading to a markedly increased expression of Beclin-1, LC3-II, and p-AMPK/AMPK. Following the administration of compound C, the effects of EA were reversed, which indicated that EA induced autophagy by increasing the activity of AMPK, thereby inhibiting the activity of mTOR (i.e., the AMPK/mTOR signaling pathway).
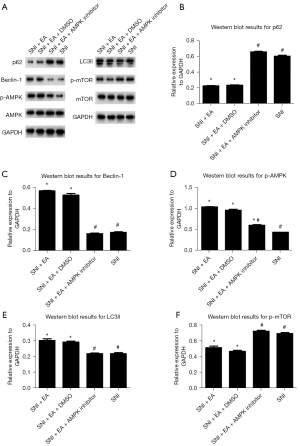
The function of the AMPK/mTOR signaling pathway in EA-induced autophagy was further evaluated by observing the autophagosomes in the microglia of the spinal dorsal horn using electron microscopy. We observed that compared to the sham group, autophagosome formation was markedly enhanced in EA-treated SNI rats, which was subsequently reversed after the administration of compound C (Figure 6). Based on these results, it can be interpreted that EA induces autophagy in the microglia of the spinal dorsal horn of SNI rats via the AMPK/mTOR signaling pathway.
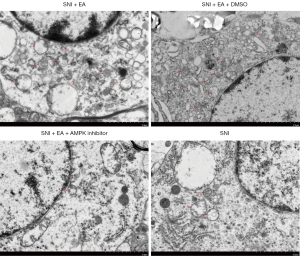
Discussion
In this investigation, the anti-nociceptive impact of EA on NP was studied, and its mechanism of action was also identified. In the dorsal horn of the spinal cord, SNI-induced upregulation of microglia was accompanied by reduced microglial autophagy. In the microglia, EA alleviated SNI-induced NP and activated autophagy. Furthermore, EA-induced suppression of NP and activation of autophagy were reversed by intrathecal administration of the AMPK inhibitor, compound C. These results indicate that EA treatment activates microglial autophagy and alleviates NP through the spinal AMPK/mTOR pathway. NP involves complex underlying mechanisms. Furthermore, the mechanism of EA induced analgesia in NP has not been completely discovered. Spinal activated microglia and the brain-derived neurotrophic factor (BDNF)-tyrosine kinase receptor B (TrkB) signaling pathway play major roles in the production and development of NP (31). EA might exert analgesic effect on NP partly through inhibiting spinal microglia activation and the BDNF-TrkB signaling pathway induced by nerve injury (31), or potentiating the function of endogenous glial cell line-derived neurotrophic factor (GDNF) and GDNF family receptor α-1 (GFRα-1, the high-affinity receptor of GDNF) system (32). Microglial autophagy might be attenuated in the spinal dorsal horn in response to SNI, which induces microglial activation, and the analgesic effect of EA could be attributed at least partially to the activation of microglial autophagy. Following nerve injury, microglial cells, which reside in the central nervous system (CNS), can switch from a resting state to an activated one. Hyperalgesia and the early stages of pain are related to microglial activation (14-16). Additionally, the activation and hypertrophy of astrocytes in the spinal cord play a vital role in the maintenance of NP (1). It has been shown that glial activation occurs in various peripheral nerve injury conditions, including chronic constriction injury of the sciatic nerve (CCI), spinal nerve ligation, tissue injury, and inflammation, all of which are associated with enhanced pain. The glial activation inhibitor relieves NP. Therefore, the inhibition of microglial activation may be considered a novel approach to treating NP.
In the present study, we investigated glial activation in SNI-induced NP rats. Our results are consistent with previous reports, which have indicated that SNI induces the activation of both astrocytes and microglia in the dorsal horn of the spinal cord, and is characterized by hyperplasia and hypertrophy. The neurons localized in the spinal dorsal horn did not change significantly following SNI. Furthermore, our double-labeling immunofluorescence results showed that p62 is expressed in nearly all microglia but not in astrocytes or neurons. We also observed that EA induced autophagy in the microglia of the dorsal horn of the spinal cord in the SNI rats, as evidenced by the results obtained from TEM. Microglial activation and hypertrophy in the presence of NP may be linked to impaired autophagy, and the enhancement of microglial autophagy may account for the positive effects of EA on NP (17,18). To improve NP, EA inhibits microglial activation by enhancing autophagy. In this study, we also examined the correlation between autophagy and the analgesic effects of EA on NP based on variations in the expression of LC3, Beclin-1, and p62 found in the spinal cord of SNI rats following EA treatment.
LC3 is an autophagic protein in mammals that is mainly found in the membranes of autophagosomes present in the cytosol. After induction of autophagy, LC3-I from the cytosol is converted into LC3-II, which occurs in the autophagosome membrane (33). The plasma membrane, cytoplasm, and nucleus contain Beclin-1, which is crucial for the localization of autophagic proteins to a pre-autophagosomal structure (34). P62 is considered a selective autophagy receptor that is primarily degraded by autophagy; thus, an increase in the p62 level corresponds to a reduction in autophagy (35). P62 has also been shown to have a connection with LC3 and ubiquitinated substrates. Moreover, it is incorporated into autophagosomes and degraded in autophagolysosomes (36).
The rats in our study exhibited significant mechanical allodynia following the induction of SNI; the expression level of p62 in the spinal cord was elevated to a significant level, indicating that autophagic flux was blocked in autophagy. Additionally, the administration of EA markedly reversed mechanical allodynia, which was accompanied by a decrease in p62 levels, indicating that EA enhanced autophagy. The expressions of LC3-II and Beclin-1 were enhanced after EA stimulation, suggesting enhanced autophagy. Compared to the sham group rats, a notable increase in the expression of Beclin-1 and LC3-II was observed in the SNI group (Figure 1D,1E). Our results indicated that autophagy was attenuated following SNI, based on the increased expression levels of p62. Elevated Beclin-1 and LC3-II levels following nerve injury may be attributable to impaired autophagosome clearance rather than enhanced autophagy. Local autophagy can sequester presynaptic components and therefore modulate presynaptic function (37) as well as selectively degrade postsynaptic receptors (38,39). In addition to the well-established role in cellular homeostasis and stress response, the emerging role of autophagy in neurotransmitter release modulation and synaptic as well dendritic remodeling may reveal important also in the context of the structural and functional changes occurring at spinal dorsal horn during chronic pain states (40,41). Impaired autophagy can be deleterious not only by failing to provide energy for essential cell functions but also by allowing accumulation of damaged cellular components and dysfunctional proteins, as well as impairing receptors trafficking and degradation (42), thus affecting neuronal activity. As a result, autophagy may play a major role in the analgesic effect of EA in NP rats (43).
The activation of the AMPK/mTOR pathway is critical for reducing chronic pain and controlling autophagic flux (20). Furthermore, AMPK expression in the hypothalamus is related to the analgesic effect of EA, and the AMPK expression levels in the responding rats are higher than those in non-responding rats (21). To investigate the role of the AMPK/mTOR pathway in EA-mediated autophagy in microglial cells, we used a pharmacological inhibitor that has the potential to inhibit AMPK activity. Dorsomorphin (compound C) is a selective, competitive inhibitor of AMPK. AMPK can activate ULK1, which plays a critical role in autophagy initiation, by directly phosphorylating ULK1 at Ser317 and Ser777 and by indirectly inactivating mTOR, which results in the dephosphorylation of ULK1 at Ser757 (44). After EA stimulation, AMPK phosphorylation was increased (Figure 5D), and mTOR phosphorylation was decreased (Figure 5F). EA significantly induced AMPK phosphorylation and attenuated mTOR phosphorylation which could be reversed by the AMPK inhibitor compound C (Figure 5D,5F). Compound C also prevented autophagosome formation in microglial cells of spinal dorsal horn (Figure 6), and prevented LC3 and Belclin-1 accumulation and p62 degradation in EA treated rats (Figure 5B,5C,5E). The data revealed that EA could induce autophagy, which could be reversed by compound C, suggesting that the activation of autophagy was regulated by AMPK and mTOR in response to peripheral nerve injury in microglial cells of spinal dorsal horn. These results provide a theoretical basis for further clinical application of the EA.
A study has reported that caspase signaling can regulate the activation and neurotoxicity of microglia (45). However, we did not take the cell culture study to explore the caspase signal in microglia, which would be the future directions of our research.
Using TEM and autophagy-related proteins, we demonstrated that inhibiting AMPK activity attenuated autophagy induction and suppressed EA-induced autophagy in microglial cells. According to our findings, AMPK/mTOR are involved in triggering autophagy in microglial cells upon EA stimulation in SNI rats. Our study provides further insight into the molecular mechanism of EA mediated analgesia in peripheral nerve injury and presents evidence for the role AMPK/mTOR signaling pathway in autophagy of spinal microglial cells induced by EA stimulation for NP. Autophagy-targeting drugs have emerged as a target in NP research. Our research provides a basis and guidance for autophagy-targeting pathway and EA application in pain therapy, and further clarifies the role and regulation AMPK/mTOR signaling pathway in EA induced analgesia, offering new targets and ideas for pain therapy. Due to the high expense, we did not take out gene knock-out experiments. The future study may be focused on target genes.
Conclusions
Our work showed that the analgesic impact of EA is partly related to AMPK/mTOR pathway activation and autophagy induction in microglial cells, providing a potential therapeutic target for NP.
Acknowledgments
We thank Zongxiang Tang (MD, School of Medicine & Holistic Integrative Medicine, Nanjing University of Chinese Medicine, Nanjing, China) and Yan Yang (MD, School of Medicine & Holistic Integrative Medicine, Nanjing University of Chinese Medicine, Nanjing, China) for their assistance with the experiments. We also thank Bullet Edits Limited for the linguistic editing and proofreading of the manuscript.
Funding: The present study was supported by the National Natural Science Foundation of China (No. 81803859) and the Natural Science Foundation of Jiangsu Province (No. BK20181096).
Footnote
Reporting Checklist: The authors have completed the ARRIVE reporting checklist. Available at https://atm.amegroups.com/article/view/10.21037/atm-22-5273/rc
Data Sharing Statement: Available at https://atm.amegroups.com/article/view/10.21037/atm-22-5273/dss
Conflicts of Interest: All authors have completed the ICMJE uniform disclosure form (available at https://atm.amegroups.com/article/view/10.21037/atm-22-5273/coif). The authors have no conflicts of interest to declare.
Ethical Statement: The authors are accountable for all aspects of the work in ensuring that questions related to the accuracy or integrity of any part of the work are appropriately investigated and resolved. Animal experiments were performed under a project license (No. ACU210502) granted by the Committee on animal experimentation at Nanjing University of Chinese Medicine, in compliance with national guidelines for the care and use of animals.
Open Access Statement: This is an Open Access article distributed in accordance with the Creative Commons Attribution-NonCommercial-NoDerivs 4.0 International License (CC BY-NC-ND 4.0), which permits the non-commercial replication and distribution of the article with the strict proviso that no changes or edits are made and the original work is properly cited (including links to both the formal publication through the relevant DOI and the license). See: https://creativecommons.org/licenses/by-nc-nd/4.0/.
References
- Zhang X, Xu Y, Wang J, et al. The effect of intrathecal administration of glial activation inhibitors on dorsal horn BDNF overexpression and hind paw mechanical allodynia in spinal nerve ligated rats. J Neural Transm (Vienna) 2012;119:329-36. [Crossref] [PubMed]
- Raghavendra V, Tanga F, DeLeo JA. Inhibition of microglial activation attenuates the development but not existing hypersensitivity in a rat model of neuropathy. J Pharmacol Exp Ther 2003;306:624-30. [Crossref] [PubMed]
- Moulin DE, Clark AJ, Gilron I, et al. Pharmacological management of chronic neuropathic pain - consensus statement and guidelines from the Canadian Pain Society. Pain Res Manag 2007;12:13-21. [Crossref] [PubMed]
- Hershman DL, Lacchetti C, Dworkin RH, et al. Prevention and management of chemotherapy-induced peripheral neuropathy in survivors of adult cancers: American Society of Clinical Oncology clinical practice guideline. J Clin Oncol 2014;32:1941-67. [Crossref] [PubMed]
- Lv ZT, Shen LL, Zhu B, et al. Effects of intensity of electroacupuncture on chronic pain in patients with knee osteoarthritis: a randomized controlled trial. Arthritis Res Ther 2019;21:120. [Crossref] [PubMed]
- Xu J, Chen L, Tang L, et al. Electroacupuncture inhibits weight gain in diet-induced obese rats by activating hypothalamic LKB1-AMPK signaling. BMC Complement Altern Med 2015;15:147. [Crossref] [PubMed]
- Zhang R, Lao L, Ren K, et al. Mechanisms of acupuncture-electroacupuncture on persistent pain. Anesthesiology 2014;120:482-503. [Crossref] [PubMed]
- Levine B, Kroemer G. Autophagy in the pathogenesis of disease. Cell 2008;132:27-42. [Crossref] [PubMed]
- Jung KT, Lim KJ. Autophagy: Can It be a New Experimental Research Method of Neuropathic Pain? Korean J Pain 2015;28:229-30. [Crossref] [PubMed]
- Mizushima N, Levine B, Cuervo AM, et al. Autophagy fights disease through cellular self-digestion. Nature 2008;451:1069-75. [Crossref] [PubMed]
- Shi G, Shi J, Liu K, et al. Increased miR-195 aggravates neuropathic pain by inhibiting autophagy following peripheral nerve injury. Glia 2013;61:504-12. [Crossref] [PubMed]
- Liu X, Zhu M, Ju Y, et al. Autophagy dysfunction in neuropathic pain. Neuropeptides 2019;75:41-8. [Crossref] [PubMed]
- Guo JS, Jing PB, Wang JA, et al. Increased autophagic activity in dorsal root ganglion attenuates neuropathic pain following peripheral nerve injury. Neurosci Lett 2015;599:158-63. [Crossref] [PubMed]
- DeLeo JA, Yezierski RP. The role of neuroinflammation and neuroimmune activation in persistent pain. Pain 2001;90:1-6. [Crossref] [PubMed]
- Watkins LR, Milligan ED, Maier SF. Glial activation: a driving force for pathological pain. Trends Neurosci 2001;24:450-5. [Crossref] [PubMed]
- Wieseler-Frank J, Maier SF, Watkins LR. Glial activation and pathological pain. Neurochem Int 2004;45:389-95. [Crossref] [PubMed]
- Shan S, Qi-Liang MY, Hong C, et al. Is functional state of spinal microglia involved in the anti-allodynic and anti-hyperalgesic effects of electroacupuncture in rat model of monoarthritis? Neurobiol Dis 2007;26:558-68. [Crossref] [PubMed]
- Sun S, Cao H, Han M, et al. Evidence for suppression of electroacupuncture on spinal glial activation and behavioral hypersensitivity in a rat model of monoarthritis. Brain Res Bull 2008;75:83-93. [Crossref] [PubMed]
- Chen W, Lu Z. Upregulated TLR3 Promotes Neuropathic Pain by Regulating Autophagy in Rat With L5 Spinal Nerve Ligation Model. Neurochem Res 2017;42:634-43. [Crossref] [PubMed]
- Melemedjian OK, Asiedu MN, Tillu DV, et al. Targeting adenosine monophosphate-activated protein kinase (AMPK) in preclinical models reveals a potential mechanism for the treatment of neuropathic pain. Mol Pain 2011;7:70. [Crossref] [PubMed]
- Kim SK, Sun B, Yoon H, et al. Expression levels of the hypothalamic AMPK gene determines the responsiveness of the rats to electroacupuncture-induced analgesia. BMC Complement Altern Med 2014;14:211. [Crossref] [PubMed]
- Cichon J, Sun L, Yang G. Spared Nerve Injury Model of Neuropathic Pain in Mice. Bio Protoc 2018;8:e2777. [Crossref] [PubMed]
- Cui WQ, Sun WS, Xu F, et al. Spinal Serotonin 1A Receptor Contributes to the Analgesia of Acupoint Catgut Embedding by Inhibiting Phosphorylation of the N-Methyl-d-Aspartate Receptor GluN1 Subunit in Complete Freund's Adjuvant-Induced Inflammatory Pain in Rats. J Pain 2019;20:16.e1-16.e16. [Crossref] [PubMed]
- Luo H, Zhang Y, Zhang J, et al. Glucocorticoid Receptor Contributes to Electroacupuncture-Induced Analgesia by Inhibiting Nav1.7 Expression in Rats With Inflammatory Pain Induced by Complete Freund's Adjuvant. Neuromodulation 2022;25:1393-402. [Crossref] [PubMed]
- Wu JJ, Lu YC, Hua XY, et al. A Longitudinal Mapping Study on Cortical Plasticity of Peripheral Nerve Injury Treated by Direct Anastomosis and Electroacupuncture in Rats. World Neurosurg 2018;114:e267-82. [Crossref] [PubMed]
- Calvo M, Zhu N, Grist J, et al. Following nerve injury neuregulin-1 drives microglial proliferation and neuropathic pain via the MEK/ERK pathway. Glia 2011;59:554-68. [Crossref] [PubMed]
- Chaplan SR, Bach FW, Pogrel JW, et al. Quantitative assessment of tactile allodynia in the rat paw. J Neurosci Methods 1994;53:55-63. [Crossref] [PubMed]
- Johansen T, Lamark T. Selective autophagy mediated by autophagic adapter proteins. Autophagy 2011;7:279-96. [Crossref] [PubMed]
- Bjørkøy G, Lamark T, Brech A, et al. p62/SQSTM1 forms protein aggregates degraded by autophagy and has a protective effect on huntingtin-induced cell death. J Cell Biol 2005;171:603-14. [Crossref] [PubMed]
- Galluzzi L, Aaronson SA, Abrams J, et al. Guidelines for the use and interpretation of assays for monitoring cell death in higher eukaryotes. Cell Death Differ 2009;16:1093-107. [Crossref] [PubMed]
- Tsuda M, Beggs S, Salter MW, et al. Microglia and intractable chronic pain. Glia 2013;61:55-61. [Crossref] [PubMed]
- Dong ZQ, Ma F, Xie H, et al. Changes of expression of glial cell line-derived neurotrophic factor and its receptor in dorsal root ganglions and spinal dorsal horn during electroacupuncture treatment in neuropathic pain rats. Neurosci Lett 2005;376:143-8. [Crossref] [PubMed]
- Chen H, Hu Y, Xie K, et al. Effect of autophagy on allodynia, hyperalgesia and astrocyte activation in a rat model of neuropathic pain. Int J Mol Med 2018;42:2009-19. [Crossref] [PubMed]
- Kang R, Zeh HJ, Lotze MT, et al. The Beclin 1 network regulates autophagy and apoptosis. Cell Death Differ 2011;18:571-80. [Crossref] [PubMed]
- Mizushima N, Hara T. Intracellular quality control by autophagy: how does autophagy prevent neurodegeneration? Autophagy 2006;2:302-4. [Crossref] [PubMed]
- Yuan J, Fei Y. Lidocaine activates autophagy of astrocytes and ameliorates chronic constriction injury-induced neuropathic pain. J Biochem 2021;170:25-31. [Crossref] [PubMed]
- Hernandez D, Torres CA, Setlik W, et al. Regulation of presynaptic neurotransmission by macroautophagy. Neuron 2012;74:277-84. [Crossref] [PubMed]
- Matsuda S, Yuzaki M. AP-4: autophagy-four mislocalized proteins in axons. Autophagy 2008;4:815-6. [Crossref] [PubMed]
- Rowland AM, Richmond JE, Olsen JG, et al. Presynaptic terminals independently regulate synaptic clustering and autophagy of GABAA receptors in Caenorhabditis elegans. J Neurosci 2006;26:1711-20. [Crossref] [PubMed]
- Kuner R. Central mechanisms of pathological pain. Nat Med 2010;16:1258-66. [Crossref] [PubMed]
- Berliocchi L, Russo R, Tassorelli C, et al. Death in pain: peripheral nerve injury and spinal neurodegenerative mechanisms. Curr Opin Pharmacol 2012;12:49-54. [Crossref] [PubMed]
- Boland B, Nixon RA. Neuronal macroautophagy: from development to degeneration. Mol Aspects Med 2006;27:503-19. [Crossref] [PubMed]
- Jin GL, Yue RC, He SD, et al. Koumine Decreases Astrocyte-Mediated Neuroinflammation and Enhances Autophagy, Contributing to Neuropathic Pain From Chronic Constriction Injury in Rats. Front Pharmacol 2018;9:989. [Crossref] [PubMed]
- Zhao Y, Hu X, Liu Y, et al. ROS signaling under metabolic stress: cross-talk between AMPK and AKT pathway. Mol Cancer 2017;16:79. [Crossref] [PubMed]
- Ali F, Hossain MS, Abdeen A, et al. Plasmalogens ensure the stability of non-neuronal (microglial) cells during long-term cytotoxicity. Environ Sci Pollut Res Int 2022;29:2084-97. [Crossref] [PubMed]
(English Language Editor: A. Kassem)